On a Possible Giant Impact Origin for the Colorado Plateau
Total Page:16
File Type:pdf, Size:1020Kb
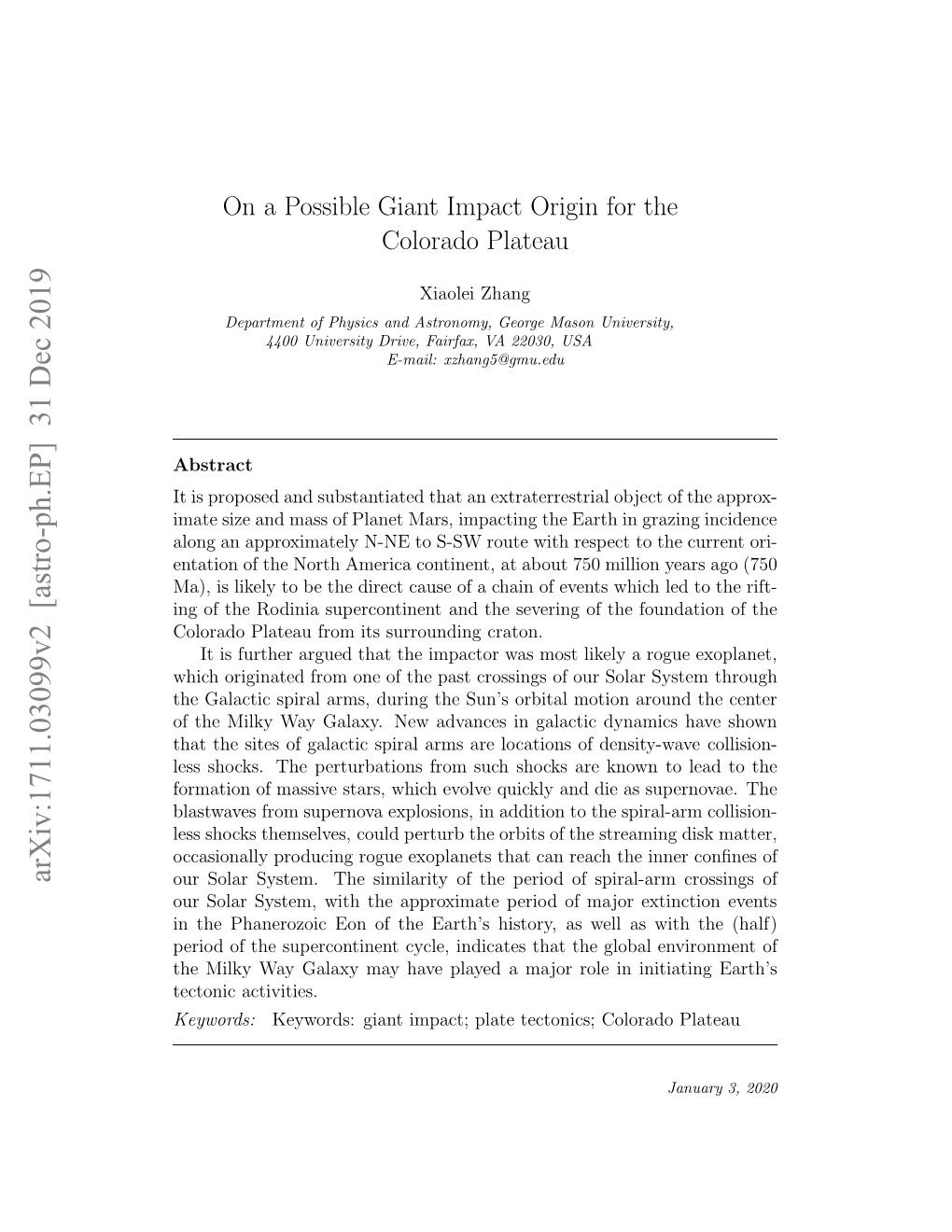
Load more
Recommended publications
-
2017 ANNUAL REPORT 2017 Annual Report Table of Contents the Michael J
Roadmaps for Progress 2017 ANNUAL REPORT 2017 Annual Report Table of Contents The Michael J. Fox Foundation is dedicated to finding a cure for 2 A Note from Michael Parkinson’s disease through an 4 Annual Letter from the CEO and the Co-Founder aggressively funded research agenda 6 Roadmaps for Progress and to ensuring the development of 8 2017 in Photos improved therapies for those living 10 2017 Donor Listing 16 Legacy Circle with Parkinson’s today. 18 Industry Partners 26 Corporate Gifts 32 Tributees 36 Recurring Gifts 39 Team Fox 40 Team Fox Lifetime MVPs 46 The MJFF Signature Series 47 Team Fox in Photos 48 Financial Highlights 54 Credits 55 Boards and Councils Milestone Markers Throughout the book, look for stories of some of the dedicated Michael J. Fox Foundation community members whose generosity and collaboration are moving us forward. 1 The Michael J. Fox Foundation 2017 Annual Report “What matters most isn’t getting diagnosed with Parkinson’s, it’s A Note from what you do next. Michael J. Fox The choices we make after we’re diagnosed Dear Friend, can open doors to One of the great gifts of my life is that I've been in a position to take my experience with Parkinson's and combine it with the perspectives and expertise of others to accelerate possibilities you’d improved treatments and a cure. never imagine.’’ In 2017, thanks to your generosity and fierce belief in our shared mission, we moved closer to this goal than ever before. For helping us put breakthroughs within reach — thank you. -
OSAA Girls Basketball Championships
OSAA Girls Basketball Championships State Champions and 2nd Place Teams Year Class Champion (W-L) Coach 2nd Place (W-L) Coach Score Site 1976 AAA South Salem (20-2) Roberta Perkins Wilson Stan Stanton 41-32 Lewis & Clark College, Portland AA Yamhill-Carlton (19-1) Greg Stiff Crook County Tim Huntley 43-40 Marshfield HS, Coos Bay A Scio (21-3) Kay Mayer Dayville Norman Hoffman 51-34 Pendleton Armory 1977 AAA Wilson (20-1) Stan Stanton St. Mary’s Kathy Kinyon 53-51 Lewis & Clark College, Portland AA Madras (25-2) Dave Wiles Rogue River Bill Willard 43-41 Marshfield HS, Coos Bay A Knappa (21-1) Renee Boak Mapleton Karen Cooper 40-39 Pendleton Armory B Long Creek (22-3) Harold Preston Paisley Maureen McElligott 45-23 Baker HS 1978 AAA Crescent Valley (24-1) Lyle Fagnan Hillsboro Dave Olmstead 54-51 Salem Armory AA Newport (24-1) Dick Mason Marist Karen & Paul Brothers 43-36 Marshfield HS, Coos Bay A Pilot Rock (23-2) Gail Scharfe Knappa Renee Boak 55-50 Pendleton Armory B Wheeler (21-3) Gail Thompson Paisley Maureen McElligott 54-50 Baker HS 1979 AAA Hillsboro (21-1) Dave Olmstead Dallas Kathy Voves 61-51 Salem Armory AA Crook County (19-0) Tim Huntley La Salle Jeff Gray 50-45 Salem Armory A Sacred Heart (27-1) Wendy Gray Pilot Rock Gail Scharfe 49-35 Pendleton Armory B Cove (23-2) Kathy Looslie Arlington Jim Turner 46-35 Baker HS 1980 AAA Hillsboro (21-1) Dave Olmstead St. Mary’s Kathy Kinyon 43-38 Salem Armory AA Henley (25-2) Dick Reiling La Salle Jeff Gray 39-36 Salem Armory A Corbett (17-6) Tom Anderson Pilot Rock Gail Scharfe 51-44 Pendleton Armory B Cove (19-4) John Ott Eddyville Steve Brattain 46-27 Baker HS 1981 AAA Marshall (26-0) Ken Trapp St. -
COURT of CLAIMS of THE
REPORTS OF Cases Argued and Determined IN THE COURT of CLAIMS OF THE STATE OF ILLINOIS VOLUME 39 Containing cases in which opinions were filed and orders of dismissal entered, without opinion for: Fiscal Year 1987 - July 1, 1986-June 30, 1987 SPRINGFIELD, ILLINOIS 1988 (Printed by authority of the State of Illinois) (65655--300-7/88) PREFACE The opinions of the Court of Claims reported herein are published by authority of the provisions of Section 18 of the Court of Claims Act, Ill. Rev. Stat. 1987, ch. 37, par. 439.1 et seq. The Court of Claims has exclusive jurisdiction to hear and determine the following matters: (a) all claims against the State of Illinois founded upon any law of the State, or upon an regulation thereunder by an executive or administrative ofgcer or agency, other than claims arising under the Workers’ Compensation Act or the Workers’ Occupational Diseases Act, or claims for certain expenses in civil litigation, (b) all claims against the State founded upon any contract entered into with the State, (c) all claims against the State for time unjustly served in prisons of this State where the persons imprisoned shall receive a pardon from the Governor stating that such pardon is issued on the grounds of innocence of the crime for which they were imprisoned, (d) all claims against the State in cases sounding in tort, (e) all claims for recoupment made by the State against any Claimant, (f) certain claims to compel replacement of a lost or destroyed State warrant, (g) certain claims based on torts by escaped inmates of State institutions, (h) certain representation and indemnification cases, (i) all claims pursuant to the Law Enforcement Officers, Civil Defense Workers, Civil Air Patrol Members, Paramedics and Firemen Compensation Act, (j) all claims pursuant to the Illinois National Guardsman’s and Naval Militiaman’s Compensation Act, and (k) all claims pursuant to the Crime Victims Compensation Act. -
DMAAC – February 1973
LUNAR TOPOGRAPHIC ORTHOPHOTOMAP (LTO) AND LUNAR ORTHOPHOTMAP (LO) SERIES (Published by DMATC) Lunar Topographic Orthophotmaps and Lunar Orthophotomaps Scale: 1:250,000 Projection: Transverse Mercator Sheet Size: 25.5”x 26.5” The Lunar Topographic Orthophotmaps and Lunar Orthophotomaps Series are the first comprehensive and continuous mapping to be accomplished from Apollo Mission 15-17 mapping photographs. This series is also the first major effort to apply recent advances in orthophotography to lunar mapping. Presently developed maps of this series were designed to support initial lunar scientific investigations primarily employing results of Apollo Mission 15-17 data. Individual maps of this series cover 4 degrees of lunar latitude and 5 degrees of lunar longitude consisting of 1/16 of the area of a 1:1,000,000 scale Lunar Astronautical Chart (LAC) (Section 4.2.1). Their apha-numeric identification (example – LTO38B1) consists of the designator LTO for topographic orthophoto editions or LO for orthophoto editions followed by the LAC number in which they fall, followed by an A, B, C or D designator defining the pertinent LAC quadrant and a 1, 2, 3, or 4 designator defining the specific sub-quadrant actually covered. The following designation (250) identifies the sheets as being at 1:250,000 scale. The LTO editions display 100-meter contours, 50-meter supplemental contours and spot elevations in a red overprint to the base, which is lithographed in black and white. LO editions are identical except that all relief information is omitted and selenographic graticule is restricted to border ticks, presenting an umencumbered view of lunar features imaged by the photographic base. -
Flynn Creek Crater, Tennessee: Final Report, by David J
1967010060 ASTROGEOLOGIC STUDIES / ANNUAL PROGRESS REPORT " July 1, 1965 to July 1, 1966 ° 'i t PART B - h . CRATERINVESTIGATIONS N 67_1_389 N 57-" .]9400 (ACCEC_ION [4U _" EiER! (THRU} .2_ / PP (PAGLS) (CO_ w ) _5 (NASA GR OR I"MX OR AD NUMBER) (_ATEGORY) DEPARTMENT OF THE INTERIOR UNITED STATES GEOLOQICAL SURVEY • iri i i i i iiii i i 1967010060-002 ASTROGEOLOGIC STUDIES ANNUAL PROGRESS REPORT July i, 1965 to July I, 1966 PART B: CRATER INVESTIGATIONS November 1966 This preliminary report is distributed without editorial and technical review for conformity with official standards and nomenclature. It should not be quoted without permission. This report concerns work done on behalf of the National Aeronautics and Space Administration. DEPARTMENT OF THE INTERIOR UNITED STATES GEOLOGICAL SURVEY 1967010060-003 • #' C OING PAGE ,BLANK NO/" FILMED. CONTENTS PART B--CRATER INVESTIGATIONS Page Introduction ........................ vii History and origin of the Flynn Creek crater, Tennessee: final report, by David J. Roddy .............. 1 Introductien ..................... 1 Geologic history of the Flynn Creek crater ....... 5 Origin of the Flynn Creek crater ............ ii Conc lusions ...................... 32 References cited .................... 35 Geology of the Sierra Madera structure, Texas: progress report, by H. G. Wilshire ............ 41_ Introduction ...................... 41 Stratigraphy ...................... 41 Petrography and chemical composition .......... 49 S truc ture ....................... 62 References cited ............. ...... 69 Some aspects of the Manicouagan Lake structure in Quebec, Canada, by Stephen H. Wolfe ................ 71 f Craters produced by missile impacts, by H. J. Moore ..... 79 Introduction ...................... 79 Experimental procedure ................. 80 Experimental results .................. 81 Summary ........................ 103 References cited .................... 103 Hypervelocity impact craters in pumice, by H. J. Moore and / F. -
Appendix I Lunar and Martian Nomenclature
APPENDIX I LUNAR AND MARTIAN NOMENCLATURE LUNAR AND MARTIAN NOMENCLATURE A large number of names of craters and other features on the Moon and Mars, were accepted by the IAU General Assemblies X (Moscow, 1958), XI (Berkeley, 1961), XII (Hamburg, 1964), XIV (Brighton, 1970), and XV (Sydney, 1973). The names were suggested by the appropriate IAU Commissions (16 and 17). In particular the Lunar names accepted at the XIVth and XVth General Assemblies were recommended by the 'Working Group on Lunar Nomenclature' under the Chairmanship of Dr D. H. Menzel. The Martian names were suggested by the 'Working Group on Martian Nomenclature' under the Chairmanship of Dr G. de Vaucouleurs. At the XVth General Assembly a new 'Working Group on Planetary System Nomenclature' was formed (Chairman: Dr P. M. Millman) comprising various Task Groups, one for each particular subject. For further references see: [AU Trans. X, 259-263, 1960; XIB, 236-238, 1962; Xlffi, 203-204, 1966; xnffi, 99-105, 1968; XIVB, 63, 129, 139, 1971; Space Sci. Rev. 12, 136-186, 1971. Because at the recent General Assemblies some small changes, or corrections, were made, the complete list of Lunar and Martian Topographic Features is published here. Table 1 Lunar Craters Abbe 58S,174E Balboa 19N,83W Abbot 6N,55E Baldet 54S, 151W Abel 34S,85E Balmer 20S,70E Abul Wafa 2N,ll7E Banachiewicz 5N,80E Adams 32S,69E Banting 26N,16E Aitken 17S,173E Barbier 248, 158E AI-Biruni 18N,93E Barnard 30S,86E Alden 24S, lllE Barringer 29S,151W Aldrin I.4N,22.1E Bartels 24N,90W Alekhin 68S,131W Becquerei -
User Guide to 1:250,000 Scale Lunar Maps
CORE https://ntrs.nasa.gov/search.jsp?R=19750010068Metadata, citation 2020-03-22T22:26:24+00:00Z and similar papers at core.ac.uk Provided by NASA Technical Reports Server USER GUIDE TO 1:250,000 SCALE LUNAR MAPS (NASA-CF-136753) USE? GJIDE TO l:i>,, :LC h75- lu1+3 SCALE LUNAR YAPS (Lumoalcs Feseclrch Ltu., Ottewa (Ontario) .) 24 p KC 53.25 CSCL ,33 'JIACA~S G3/31 11111 DANNY C, KINSLER Lunar Science Instltute 3303 NASA Road $1 Houston, TX 77058 Telephone: 7131488-5200 Cable Address: LUtiSI USER GUIDE TO 1: 250,000 SCALE LUNAR MAPS GENERAL In 1972 the NASA Lunar Programs Office initiated the Apollo Photographic Data Analysis Program. The principal point of this program was a detailed scientific analysis of the orbital and surface experiments data derived from Apollo missions 15, 16, and 17. One of the requirements of this program was the production of detailed photo base maps at a useable scale. NASA in conjunction with the Defense Mapping Agency (DMA) commenced a mapping program in early 1973 that would lead to the production of the necessary maps based on the need for certain areas. This paper is designed to present in outline form the neces- sary background informatiox or users to become familiar with the program. MAP FORMAT * The scale chosen for the project was 1:250,000 . The re- search being done required a scale that Principal Investigators (PI'S) using orbital photography could use, but would also serve PI'S doing surface photographic investigations. Each map sheet covers an area four degrees north/south by five degrees east/west. -
The Tennessee Meteorite Impact Sites and Changing Perspectives on Impact Cratering
UNIVERSITY OF SOUTHERN QUEENSLAND THE TENNESSEE METEORITE IMPACT SITES AND CHANGING PERSPECTIVES ON IMPACT CRATERING A dissertation submitted by Janaruth Harling Ford B.A. Cum Laude (Vanderbilt University), M. Astron. (University of Western Sydney) For the award of Doctor of Philosophy 2015 ABSTRACT Terrestrial impact structures offer astronomers and geologists opportunities to study the impact cratering process. Tennessee has four structures of interest. Information gained over the last century and a half concerning these sites is scattered throughout astronomical, geological and other specialized scientific journals, books, and literature, some of which are elusive. Gathering and compiling this widely- spread information into one historical document benefits the scientific community in general. The Wells Creek Structure is a proven impact site, and has been referred to as the ‘syntype’ cryptoexplosion structure for the United State. It was the first impact structure in the United States in which shatter cones were identified and was probably the subject of the first detailed geological report on a cryptoexplosive structure in the United States. The Wells Creek Structure displays bilateral symmetry, and three smaller ‘craters’ lie to the north of the main Wells Creek structure along its axis of symmetry. The question remains as to whether or not these structures have a common origin with the Wells Creek structure. The Flynn Creek Structure, another proven impact site, was first mentioned as a site of disturbance in Safford’s 1869 report on the geology of Tennessee. It has been noted as the terrestrial feature that bears the closest resemblance to a typical lunar crater, even though it is the probable result of a shallow marine impact. -
Summary of Sexual Abuse Claims in Chapter 11 Cases of Boy Scouts of America
Summary of Sexual Abuse Claims in Chapter 11 Cases of Boy Scouts of America There are approximately 101,135sexual abuse claims filed. Of those claims, the Tort Claimants’ Committee estimates that there are approximately 83,807 unique claims if the amended and superseded and multiple claims filed on account of the same survivor are removed. The summary of sexual abuse claims below uses the set of 83,807 of claim for purposes of claims summary below.1 The Tort Claimants’ Committee has broken down the sexual abuse claims in various categories for the purpose of disclosing where and when the sexual abuse claims arose and the identity of certain of the parties that are implicated in the alleged sexual abuse. Attached hereto as Exhibit 1 is a chart that shows the sexual abuse claims broken down by the year in which they first arose. Please note that there approximately 10,500 claims did not provide a date for when the sexual abuse occurred. As a result, those claims have not been assigned a year in which the abuse first arose. Attached hereto as Exhibit 2 is a chart that shows the claims broken down by the state or jurisdiction in which they arose. Please note there are approximately 7,186 claims that did not provide a location of abuse. Those claims are reflected by YY or ZZ in the codes used to identify the applicable state or jurisdiction. Those claims have not been assigned a state or other jurisdiction. Attached hereto as Exhibit 3 is a chart that shows the claims broken down by the Local Council implicated in the sexual abuse. -
ANIC IMPACTS: MS and IRONMENTAL P ONS Abstracts Edited by Rainer Gersonde and Alexander Deutsch
ANIC IMPACTS: MS AND IRONMENTAL P ONS APRIL 15 - APRIL 17, 1999 Alfred Wegener Institute for Polar and Marine Research Bremerhaven, Germany Abstracts Edited by Rainer Gersonde and Alexander Deutsch Ber. Polarforsch. 343 (1999) ISSN 01 76 - 5027 Preface .......3 Acknowledgements .......6 Program ....... 7 Abstracts P. Agrinier, A. Deutsch, U. Schäre and I. Martinez: On the kinetics of reaction of CO, with hot Ca0 during impact events: An experimental study. .11 L. Ainsaar and M. Semidor: Long-term effect of the Kärdl impact crater (Hiiumaa, Estonia) On the middle Ordovician carbonate sedimentation. ......13 N. Artemieva and V.Shuvalov: Shock zones on the ocean floor - Numerical simulations. ......16 H. Bahlburg and P. Claeys: Tsunami deposit or not: The problem of interpreting the siliciclastic K/T sections in northeastern Mexico. ......19 R. Coccioni, D. Basso, H. Brinkhuis, S. Galeotti, S. Gardin, S. Monechi, E. Morettini, M. Renard, S. Spezzaferri, and M. van der Hoeven: Environmental perturbation following a late Eocene impact event: Evidence from the Massignano Section, Italy. ......21 I von Dalwigk and J. Ormö Formation of resurge gullies at impacts at sea: the Lockne crater, Sweden. ......24 J. Ebbing, P. Janle, J, Koulouris and B. Milkereit: Palaeotopography of the Chicxulub impact crater and implications for oceanic craters. .25 V. Feldman and S.Kotelnikov: The methods of shock pressure estimation in impacted rocks. ......28 J.-A. Flores, F. J. Sierro and R. Gersonde: Calcareous plankton stratigraphies from the "Eltanin" asteroid impact area: Strategies for geological and paleoceanographic reconstruction. ......29 M.V.Gerasimov, Y. P. Dikov, 0 . I. Yakovlev and F.Wlotzka: Experimental investigation of the role of water in the impact vaporization chemistry. -
Meteor Crater, Arizona
Petrographic, X-ray diffraction, and electron spin resonance analysis of deformed calcite: Meteor Crater, Arizona Item Type Article; text Authors Burt, J. B.; Pope, M. C.; Watkinson, A. J. Citation Burt, J. B., Pope, M. C., & Watkinson, A. J. (2005). Petrographic, Xray diffraction, and electron spin resonance analysis of deformed calcite: Meteor Crater, Arizona. Meteoritics & Planetary Science, 40(2), 297-306. DOI 10.1111/j.1945-5100.2005.tb00381.x Publisher The Meteoritical Society Journal Meteoritics & Planetary Science Rights Copyright © The Meteoritical Society Download date 05/10/2021 06:22:06 Item License http://rightsstatements.org/vocab/InC/1.0/ Version Final published version Link to Item http://hdl.handle.net/10150/655967 Meteoritics & Planetary Science 40, Nr 2, 297–306 (2005) Abstract available online at http://meteoritics.org Petrographic, X-ray diffraction, and electron spin resonance analysis of deformed calcite: Meteor Crater, Arizona Jason B. BURT,1†* Mike C. POPE,1 and A. John WATKINSON1 1Department of Geological Sciences, Washington State University, Pullman, Washington 99164, USA †Present address: Department of Geosciences, Virginia Polytechnic and State University, Blacksburg, Virginia 24061, USA *Corresponding author. E-mail: [email protected] (Received 9 June 2003; revision accepted 16 December 2004) Abstract–Calcite crystals within the Kaibab limestone in Meteor Crater, Arizona, are examined to understand how calcite is deformed during a meteorite impact. The Kaibab limestone is a silty fine- grained and fossiliferous dolomudstone and the calcite crystals occur as replaced evaporite nodules with impact-induced twinning. Twinning in the calcite is variable with deformational regimes based on abundances of crystals with twins and twin densities within crystals. -
Meteorite Impacts, Earth, and the Solar System
Traces of Catastrophe A Handbook of Shock-Metamorphic Effects in Terrestrial Meteorite Impact Structures Bevan M. French Research Collaborator Department of Mineral Sciences, MRC-119 Smithsonian Institution Washington DC 20560 LPI Contribution No. 954 i Copyright © 1998 by LUNAR AND PLANETARY INSTITUTE The Institute is operated by the Universities Space Research Association under Contract No. NASW-4574 with the National Aeronautics and Space Administration. Material in this volume may be copied without restraint for library, abstract service, education, or personal research purposes; however, republication of any portion thereof requires the written permission of the Insti- tute as well as the appropriate acknowledgment of this publication. Figures 3.1, 3.2, and 3.5 used by permission of the publisher, Oxford University Press, Inc. Figures 3.13, 4.16, 4.28, 4.32, and 4.33 used by permission of the publisher, Springer-Verlag. Figure 4.25 used by permission of the publisher, Yale University. Figure 5.1 used by permission of the publisher, Geological Society of America. See individual captions for reference citations. This volume may be cited as French B. M. (1998) Traces of Catastrophe:A Handbook of Shock-Metamorphic Effects in Terrestrial Meteorite Impact Structures. LPI Contribution No. 954, Lunar and Planetary Institute, Houston. 120 pp. This volume is distributed by ORDER DEPARTMENT Lunar and Planetary Institute 3600 Bay Area Boulevard Houston TX 77058-1113, USA Phone:281-486-2172 Fax:281-486-2186 E-mail:[email protected] Mail order requestors will be invoiced for the cost of shipping and handling. Cover Art.“One Minute After the End of the Cretaceous.” This artist’s view shows the ancestral Gulf of Mexico near the present Yucatán peninsula as it was 65 m.y.