An Expression Screen Reveals Modulators of Class II Histone Deacetylase Phosphorylation
Total Page:16
File Type:pdf, Size:1020Kb
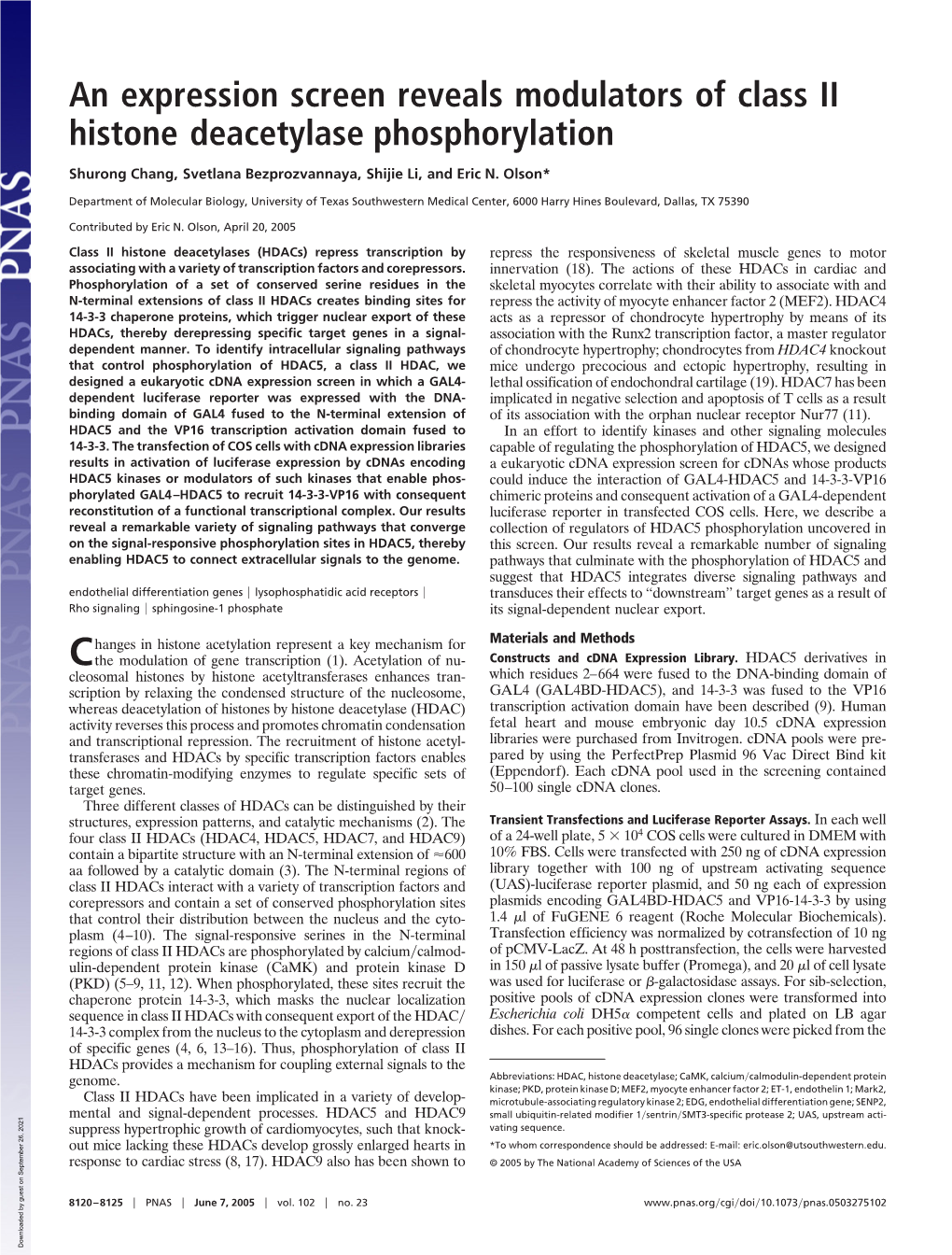
Load more
Recommended publications
-
CD40 Signaling Synergizes with TLR-2 in the BCR Independent Activation of Resting B Cells
CD40 Signaling Synergizes with TLR-2 in the BCR Independent Activation of Resting B Cells Shweta Jain, Sathi Babu Chodisetti, Javed N. Agrewala* Immunology Laboratory, Institute of Microbial Technology, Council of Scientific and Industrial Research, Chandigarh, India Abstract Conventionally, signaling through BCR initiates sequence of events necessary for activation and differentiation of B cells. We report an alternative approach, independent of BCR, for stimulating resting B (RB) cells, by involving TLR-2 and CD40 - molecules crucial for innate and adaptive immunity. CD40 triggering of TLR-2 stimulated RB cells significantly augments their activation, proliferation and differentiation. It also substantially ameliorates the calcium flux, antigen uptake capacity and ability of B cells to activate T cells. The survival of RB cells was improved and it increases the number of cells expressing activation induced deaminase (AID), signifying class switch recombination (CSR). Further, we also observed increased activation rate and decreased threshold period required for optimum stimulation of RB cells. These results corroborate well with microarray gene expression data. This study provides novel insights into coordination between the molecules of innate and adaptive immunity in activating B cells, in a BCR independent manner. This strategy can be exploited to design vaccines to bolster B cell activation and antigen presenting efficiency, leading to faster and better immune response. Citation: Jain S, Chodisetti SB, Agrewala JN (2011) CD40 Signaling Synergizes with TLR-2 in the BCR Independent Activation of Resting B Cells. PLoS ONE 6(6): e20651. doi:10.1371/journal.pone.0020651 Editor: Leonardo A. Sechi, Universita di Sassari, Italy Received April 14, 2011; Accepted May 6, 2011; Published June 2, 2011 Copyright: ß 2011 Jain et al. -
An Overview of the Role of Hdacs in Cancer Immunotherapy
International Journal of Molecular Sciences Review Immunoepigenetics Combination Therapies: An Overview of the Role of HDACs in Cancer Immunotherapy Debarati Banik, Sara Moufarrij and Alejandro Villagra * Department of Biochemistry and Molecular Medicine, School of Medicine and Health Sciences, The George Washington University, 800 22nd St NW, Suite 8880, Washington, DC 20052, USA; [email protected] (D.B.); [email protected] (S.M.) * Correspondence: [email protected]; Tel.: +(202)-994-9547 Received: 22 March 2019; Accepted: 28 April 2019; Published: 7 May 2019 Abstract: Long-standing efforts to identify the multifaceted roles of histone deacetylase inhibitors (HDACis) have positioned these agents as promising drug candidates in combatting cancer, autoimmune, neurodegenerative, and infectious diseases. The same has also encouraged the evaluation of multiple HDACi candidates in preclinical studies in cancer and other diseases as well as the FDA-approval towards clinical use for specific agents. In this review, we have discussed how the efficacy of immunotherapy can be leveraged by combining it with HDACis. We have also included a brief overview of the classification of HDACis as well as their various roles in physiological and pathophysiological scenarios to target key cellular processes promoting the initiation, establishment, and progression of cancer. Given the critical role of the tumor microenvironment (TME) towards the outcome of anticancer therapies, we have also discussed the effect of HDACis on different components of the TME. We then have gradually progressed into examples of specific pan-HDACis, class I HDACi, and selective HDACis that either have been incorporated into clinical trials or show promising preclinical effects for future consideration. -
Determining HDAC8 Substrate Specificity by Noah Ariel Wolfson A
Determining HDAC8 substrate specificity by Noah Ariel Wolfson A dissertation submitted in partial fulfillment of the requirements for the degree of Doctor of Philosophy (Biological Chemistry) in the University of Michigan 2014 Doctoral Committee: Professor Carol A. Fierke, Chair Professor Robert S. Fuller Professor Anna K. Mapp Associate Professor Patrick J. O’Brien Associate Professor Raymond C. Trievel Dedication My thesis is dedicated to all my family, mentors, and friends who made getting to this point possible. ii Table of Contents Dedication ....................................................................................................................................... ii List of Figures .............................................................................................................................. viii List of Tables .................................................................................................................................. x List of Appendices ......................................................................................................................... xi Abstract ......................................................................................................................................... xii Chapter 1 HDAC8 substrates: Histones and beyond ...................................................................... 1 Overview ..................................................................................................................................... 1 HDAC introduction -
The Metabolic Regulator Histone Deacetylase 9 Contributes to Glucose Homeostasis
Page 1 of 53 Diabetes The metabolic regulator histone deacetylase 9 contributes to glucose homeostasis abnormality induced by hepatitis C virus infection Jizheng CHEN2, Ning WANG1, Mei DONG1, Min GUO2, Yang ZHAO3, Zhiyong ZHUO3, Chao ZHANG3, Xiumei CHI4, Yu PAN4, Jing JIANG4, Hong TANG2, Junqi NIU4, Dongliang YANG5, Zhong LI1, Xiao HAN1, Qian WANG1* and Xinwen Chen2 1 Jiangsu Province Key Lab of Human Functional Genomics, Department of Biochemistry and Molecular Biology, Nanjing Medical University, Nanjing, 210029, China 2 State Key Lab of Virology, Wuhan Institute of Virology, Chinese Academy of Sciences, Wuhan, 430071, China 3 Key Laboratory of Infection and Immunity, Institute of Biophysics, Chinese Academy of Sciences, Beijing, 100101, China 4 Department of Hepatology, The First Hospital of Jilin University, Changchun, 130021, China 5 Department of Infectious Diseases, Union Hospital, Tongji Medical College, Huazhong University of Science and Technology, Wuhan, 430074, China 1 Diabetes Publish Ahead of Print, published online September 29, 2015 Diabetes Page 2 of 53 *Correspondence: Qian WANG, Ph.D. Jiangsu Province Key Lab of Human Functional Genomics Department of Biochemistry and Molecular Biology Nanjing Medical University Nanjing 210029, China E-mail: [email protected] Fax: +86-25-8362 1065 Tel: +86-25-8686 2729 2 Page 3 of 53 Diabetes ABSTRACT Class IIa histone deacetylases (HDACs), such as HDAC4, HDAC5, and HDAC7 provide critical mechanisms for regulating glucose homeostasis. Here we report HDAC9, another class IIa HDAC, regulates hepatic gluconeogenesis via deacetylation of a Forkhead box O (FoxO) family transcription factor, FoxO1, together with HDAC3. Specifically, HDAC9 expression can be strongly induced upon hepatitis C virus (HCV) infection. -
Deletion of Stk11 and Fos in Mouse BLA Projection Neurons
RESEARCH ARTICLE Deletion of Stk11 and Fos in mouse BLA projection neurons alters intrinsic excitability and impairs formation of long- term aversive memory David Levitan1†*, Chenghao Liu1†, Tracy Yang1, Yasuyuki Shima1, Jian-You Lin2,3, Joseph Wachutka2, Yasmin Marrero2, Ramin Ali Marandi Ghoddousi1, Eduardo da Veiga Beltrame2, Troy A Richter1, Donald B Katz2,3, Sacha B Nelson1,3* 1Departments of Biology, Brandeis University, Waltham, United States; 2Departments of Psychology, Brandeis University, Waltham, United States; 3Volen Center for Complex Systems, Brandeis University, Waltham, United States Abstract Conditioned taste aversion (CTA) is a form of one-trial learning dependent on basolateral amygdala projection neurons (BLApn). Its underlying cellular and molecular mechanisms remain poorly understood. RNAseq from BLApn identified changes in multiple candidate learning- related transcripts including the expected immediate early gene Fos and Stk11, a master kinase of the AMP-related kinase pathway with important roles in growth, metabolism and development, but not previously implicated in learning. Deletion of Stk11 in BLApn blocked memory prior to training, but not following it and increased neuronal excitability. Conversely, BLApn had reduced excitability following CTA. BLApn knockout of a second learning-related gene, Fos, also increased excitability and impaired learning. Independently increasing BLApn excitability chemogenetically during CTA *For correspondence: also impaired memory. STK11 and C-FOS activation were independent -
Supplementary Table S4. FGA Co-Expressed Gene List in LUAD
Supplementary Table S4. FGA co-expressed gene list in LUAD tumors Symbol R Locus Description FGG 0.919 4q28 fibrinogen gamma chain FGL1 0.635 8p22 fibrinogen-like 1 SLC7A2 0.536 8p22 solute carrier family 7 (cationic amino acid transporter, y+ system), member 2 DUSP4 0.521 8p12-p11 dual specificity phosphatase 4 HAL 0.51 12q22-q24.1histidine ammonia-lyase PDE4D 0.499 5q12 phosphodiesterase 4D, cAMP-specific FURIN 0.497 15q26.1 furin (paired basic amino acid cleaving enzyme) CPS1 0.49 2q35 carbamoyl-phosphate synthase 1, mitochondrial TESC 0.478 12q24.22 tescalcin INHA 0.465 2q35 inhibin, alpha S100P 0.461 4p16 S100 calcium binding protein P VPS37A 0.447 8p22 vacuolar protein sorting 37 homolog A (S. cerevisiae) SLC16A14 0.447 2q36.3 solute carrier family 16, member 14 PPARGC1A 0.443 4p15.1 peroxisome proliferator-activated receptor gamma, coactivator 1 alpha SIK1 0.435 21q22.3 salt-inducible kinase 1 IRS2 0.434 13q34 insulin receptor substrate 2 RND1 0.433 12q12 Rho family GTPase 1 HGD 0.433 3q13.33 homogentisate 1,2-dioxygenase PTP4A1 0.432 6q12 protein tyrosine phosphatase type IVA, member 1 C8orf4 0.428 8p11.2 chromosome 8 open reading frame 4 DDC 0.427 7p12.2 dopa decarboxylase (aromatic L-amino acid decarboxylase) TACC2 0.427 10q26 transforming, acidic coiled-coil containing protein 2 MUC13 0.422 3q21.2 mucin 13, cell surface associated C5 0.412 9q33-q34 complement component 5 NR4A2 0.412 2q22-q23 nuclear receptor subfamily 4, group A, member 2 EYS 0.411 6q12 eyes shut homolog (Drosophila) GPX2 0.406 14q24.1 glutathione peroxidase -
(P -Value<0.05, Fold Change≥1.4), 4 Vs. 0 Gy Irradiation
Table S1: Significant differentially expressed genes (P -Value<0.05, Fold Change≥1.4), 4 vs. 0 Gy irradiation Genbank Fold Change P -Value Gene Symbol Description Accession Q9F8M7_CARHY (Q9F8M7) DTDP-glucose 4,6-dehydratase (Fragment), partial (9%) 6.70 0.017399678 THC2699065 [THC2719287] 5.53 0.003379195 BC013657 BC013657 Homo sapiens cDNA clone IMAGE:4152983, partial cds. [BC013657] 5.10 0.024641735 THC2750781 Ciliary dynein heavy chain 5 (Axonemal beta dynein heavy chain 5) (HL1). 4.07 0.04353262 DNAH5 [Source:Uniprot/SWISSPROT;Acc:Q8TE73] [ENST00000382416] 3.81 0.002855909 NM_145263 SPATA18 Homo sapiens spermatogenesis associated 18 homolog (rat) (SPATA18), mRNA [NM_145263] AA418814 zw01a02.s1 Soares_NhHMPu_S1 Homo sapiens cDNA clone IMAGE:767978 3', 3.69 0.03203913 AA418814 AA418814 mRNA sequence [AA418814] AL356953 leucine-rich repeat-containing G protein-coupled receptor 6 {Homo sapiens} (exp=0; 3.63 0.0277936 THC2705989 wgp=1; cg=0), partial (4%) [THC2752981] AA484677 ne64a07.s1 NCI_CGAP_Alv1 Homo sapiens cDNA clone IMAGE:909012, mRNA 3.63 0.027098073 AA484677 AA484677 sequence [AA484677] oe06h09.s1 NCI_CGAP_Ov2 Homo sapiens cDNA clone IMAGE:1385153, mRNA sequence 3.48 0.04468495 AA837799 AA837799 [AA837799] Homo sapiens hypothetical protein LOC340109, mRNA (cDNA clone IMAGE:5578073), partial 3.27 0.031178378 BC039509 LOC643401 cds. [BC039509] Homo sapiens Fas (TNF receptor superfamily, member 6) (FAS), transcript variant 1, mRNA 3.24 0.022156298 NM_000043 FAS [NM_000043] 3.20 0.021043295 A_32_P125056 BF803942 CM2-CI0135-021100-477-g08 CI0135 Homo sapiens cDNA, mRNA sequence 3.04 0.043389246 BF803942 BF803942 [BF803942] 3.03 0.002430239 NM_015920 RPS27L Homo sapiens ribosomal protein S27-like (RPS27L), mRNA [NM_015920] Homo sapiens tumor necrosis factor receptor superfamily, member 10c, decoy without an 2.98 0.021202829 NM_003841 TNFRSF10C intracellular domain (TNFRSF10C), mRNA [NM_003841] 2.97 0.03243901 AB002384 C6orf32 Homo sapiens mRNA for KIAA0386 gene, partial cds. -
Deletion of Stk11 and Fos in Mouse BLA Projection Neurons Alters Intrinsic 3 Excitability and Impairs Formation of Long-Term Aversive Memory
bioRxiv preprint doi: https://doi.org/10.1101/787325; this version posted November 2, 2019. The copyright holder for this preprint (which was not certified by peer review) is the author/funder, who has granted bioRxiv a license to display the preprint in perpetuity. It is made available under aCC-BY-NC-ND 4.0 International license. 1 2 Deletion of Stk11 and Fos in mouse BLA projection neurons alters intrinsic 3 excitability and impairs formation of long-term aversive memory 4 5 6 Abbreviated Title: The role of Stk11 in memory formation 7 8 David Levitan2*, Chenghao Liu2*, Tracy Yang2, Yasuyuki Shima2, Jian-You Lin1, 3, Joseph 9 Wachutka1, Yasmin Marrero1, Ramin Ali Marandi Ghoddousi2, Eduardo da Veiga Beltrame1, 10 Donald B. Katz1, 3, Sacha B. Nelson2, 3 Departments of Psychology1, Biology2, and Volen Center for Complex Systems3, Brandeis University, Waltham, Massachusetts 02454, USA. 11 12 13 *D.L. and C.L. contributed equally 14 Proofs and correspondence to: 15 Sacha B Nelson, MD, PhD, Brandeis University 16 Department of Biology, MS 062, 415 South Street, Waltham, MA 02454, 17 Email: [email protected] 18 19 Declaration of Interest: The authors declare no competing financial interests. 20 Acknowledgements: This research was supported by the National Institute on Deafness and 21 Other Communication Disorders (NIDCD) DC006666 and by NINDS NS109916. 22 23 24 25 26 bioRxiv preprint doi: https://doi.org/10.1101/787325; this version posted November 2, 2019. The copyright holder for this preprint (which was not certified by peer review) is the author/funder, who has granted bioRxiv a license to display the preprint in perpetuity. -
Genetic Testing Policy Number: PG0041 ADVANTAGE | ELITE | HMO Last Review: 04/11/2021
Genetic Testing Policy Number: PG0041 ADVANTAGE | ELITE | HMO Last Review: 04/11/2021 INDIVIDUAL MARKETPLACE | PROMEDICA MEDICARE PLAN | PPO GUIDELINES This policy does not certify benefits or authorization of benefits, which is designated by each individual policyholder terms, conditions, exclusions and limitations contract. It does not constitute a contract or guarantee regarding coverage or reimbursement/payment. Paramount applies coding edits to all medical claims through coding logic software to evaluate the accuracy and adherence to accepted national standards. This medical policy is solely for guiding medical necessity and explaining correct procedure reporting used to assist in making coverage decisions and administering benefits. SCOPE X Professional X Facility DESCRIPTION A genetic test is the analysis of human DNA, RNA, chromosomes, proteins, or certain metabolites in order to detect alterations related to a heritable or acquired disorder. This can be accomplished by directly examining the DNA or RNA that makes up a gene (direct testing), looking at markers co-inherited with a disease-causing gene (linkage testing), assaying certain metabolites (biochemical testing), or examining the chromosomes (cytogenetic testing). Clinical genetic tests are those in which specimens are examined and results reported to the provider or patient for the purpose of diagnosis, prevention or treatment in the care of individual patients. Genetic testing is performed for a variety of intended uses: Diagnostic testing (to diagnose disease) Predictive -
The Role of Post-Translational Acetylation and Deacetylation of Signaling Proteins and Transcription Factors After Cerebral Ischemia: Facts and Hypotheses
International Journal of Molecular Sciences Review The Role of Post-Translational Acetylation and Deacetylation of Signaling Proteins and Transcription Factors after Cerebral Ischemia: Facts and Hypotheses Svetlana Demyanenko 1,* and Svetlana Sharifulina 1,2 1 Laboratory of Molecular Neurobiology, Academy of Biology and Biotechnology, Southern Federal University, pr. Stachki 194/1, 344090 Rostov-on-Don, Russia; [email protected] 2 Neuroscience Center HiLife, University of Helsinki, Haartmaninkatu 8, P.O. Box 63, 00014 Helsinki, Finland * Correspondence: [email protected]; Tel.: +7-918-5092185; Fax: +7-863-2230837 Abstract: Histone deacetylase (HDAC) and histone acetyltransferase (HAT) regulate transcription and the most important functions of cells by acetylating/deacetylating histones and non-histone proteins. These proteins are involved in cell survival and death, replication, DNA repair, the cell cycle, and cell responses to stress and aging. HDAC/HAT balance in cells affects gene expression and cell signaling. There are very few studies on the effects of stroke on non-histone protein acetylation/deacetylation in brain cells. HDAC inhibitors have been shown to be effective in protecting the brain from ischemic damage. However, the role of different HDAC isoforms in the survival and death of brain cells after stroke is still controversial. HAT/HDAC activity depends on the acetylation site and the acetylation/deacetylation of the main proteins (c-Myc, E2F1, p53, Citation: Demyanenko, S.; ERK1/2, Akt) considered in this review, that are involved in the regulation of cell fate decisions. Sharifulina, S. The Role of Post-Translational Acetylation and Our review aims to analyze the possible role of the acetylation/deacetylation of transcription factors Deacetylation of Signaling Proteins and signaling proteins involved in the regulation of survival and death in cerebral ischemia. -
Alzheimer's Disease As a Chronic Maladaptive Polyamine Stress
Preprints (www.preprints.org) | NOT PEER-REVIEWED | Posted: 17 February 2021 doi:10.20944/preprints202102.0362.v1 Alzheimer’s disease as a chronic maladaptive polyamine stress response Review Baruh Polis1, David Karasik2,3, Abraham O. Samson1 1 Drug Discovery Laboratory, The Azrieli Faculty of Medicine, Bar-Ilan University, Safed, 1311502, Israel. 2 Hebrew SeniorLife, Hinda and Arthur Marcus Institute for Aging Research, Boston, MA, 02131, USA 3 Musculoskeletal Genetics Laboratory, The Azrieli Faculty of Medicine, Bar-Ilan University, Safed, 1311502, Israel. Correspondence to Baruh Polis [email protected] Keywords: polyamines; arginase; senescence; aging; neurodegeneration. 1 © 2021 by the author(s). Distributed under a Creative Commons CC BY license. Preprints (www.preprints.org) | NOT PEER-REVIEWED | Posted: 17 February 2021 doi:10.20944/preprints202102.0362.v1 Abstract Polyamines are nitrogen-rich polycationic ubiquitous bioactive molecules with diverse evolutionary-conserved functions. Their activity interferes with numerous genes' expression resulting in cell proliferation and signaling modulation. The intracellular levels of polyamines are precisely controlled by evolutionary-conserved machinery. Their transient synthesis is induced by heat stress, radiation, and other traumatic stimuli in a process termed the polyamine stress response (PSR). Notably, polyamine levels decline gradually with age; and external supplementation improves lifespan in model organisms. This corresponds to cytoprotective and reactive oxygen species scavenging properties of polyamines. Paradoxically, age-associated neurodegenerative disorders are characterized by an upsurge in polyamine levels, indicating polyamine pleiotropic, adaptive, and pathogenic roles. Specifically, arginase overactivation and arginine brain deprivation have been shown to play an important role in Alzheimer’s disease (AD) pathogenesis. Here, we assert that a universal short-term PSR associated with acute stimuli is beneficial for survival. -
Impaired Autophagy Triggered by HDAC9 in Mesenchymal Stem Cells Accelerates Bone Mass Loss
Impaired autophagy triggered by HDAC9 in mesenchymal stem cells accelerates bone mass loss Running title: HDAC9 impairs MSCs differentiation via autophagy. Zhang Liqiang1,2,4, Qi Meng1, 4, Chen Ji1,4,Zhao Jiangdong3, Li Liya2, Hu Jiachen1, Jin Yan1,2*, Liu Wenjia1,2* 1. State Key Laboratory of Military Stomatology& National Clinical Research Center for Oral Diseases&Shaanxi International Joint Research Center for Oral Diseases, Center for Tissue Engineering, School of Stomatology, The Fourth Military Medical University, Xi'an, Shaanxi 710032, China. 2. Xi'an Institute of Tissue Engineering and Regenerative Medicine, Xi'an, Shaanxi 710032, China. 3. The Key Laboratory of Aerospace Medicine, Ministry of Education, The Fourth Military Medical University, Xi'an, Shaanxi 710032, China. 4. These authors contributed equally to this work. Correspondence Wenjia Liu & Yan Jin, Center for Tissue Engineering, Fourth Military Medical University, No. 145 West Changle Road, Xi’an, Shaanxi, China. Emails: [email protected] or [email protected];[email protected]; Abstract Background: Bone mass loss in aging is linked with imbalanced lineage differentiation of bone marrow mesenchymal stem cells (BMMSCs). Recent studies have proved that histone deacetylases (HDACs) are regarded as key regulators of bone remodeling. However, HDACs involve in regulating BMMSCs bio-behaviors remain elusive. Here, we investigated ability of HDAC9 on modulation of autophagy and its significance in lineage differentiation of BMMSCs. Methods: The effects of HDAC9 on lineage differentiation of BMMSCs and autophagic signalling were assessed by various biochemical (Western blot and ChIP assay), morphological (TEM and confocal microscopy) and microCT assays. Results : 16-month mice manifested obvious bone mass loss and marrow fat increase, accompanied with the decreased osteogenic differentiation and increased adipogenic differentiation of BMMSCs.