New Antimicrobial Strategies Based on Metal Complexes
Total Page:16
File Type:pdf, Size:1020Kb
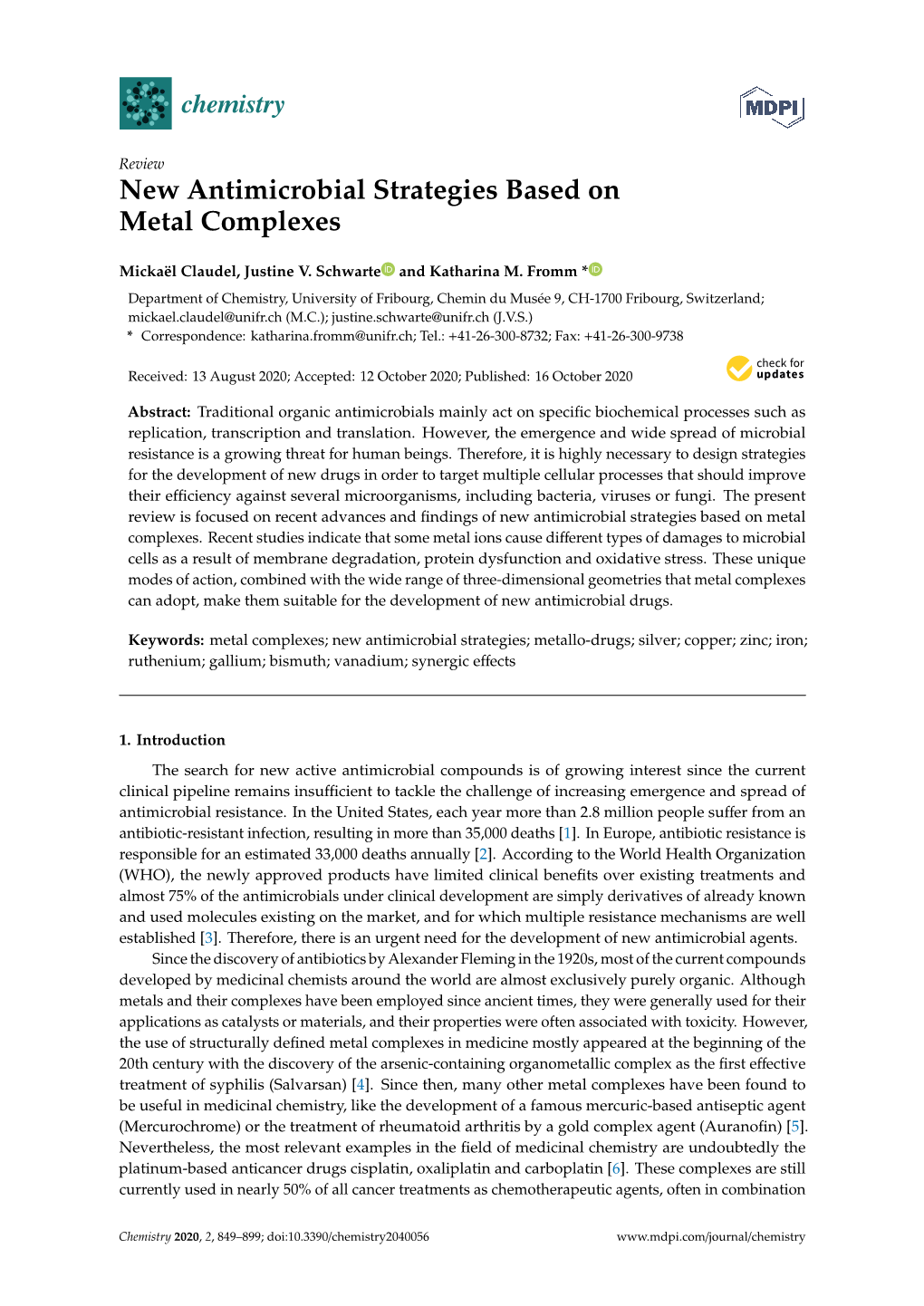
Load more
Recommended publications
-
PERFORMED IDENTITIES: HEAVY METAL MUSICIANS BETWEEN 1984 and 1991 Bradley C. Klypchak a Dissertation Submitted to the Graduate
PERFORMED IDENTITIES: HEAVY METAL MUSICIANS BETWEEN 1984 AND 1991 Bradley C. Klypchak A Dissertation Submitted to the Graduate College of Bowling Green State University in partial fulfillment of the requirements for the degree of DOCTOR OF PHILOSOPHY May 2007 Committee: Dr. Jeffrey A. Brown, Advisor Dr. John Makay Graduate Faculty Representative Dr. Ron E. Shields Dr. Don McQuarie © 2007 Bradley C. Klypchak All Rights Reserved iii ABSTRACT Dr. Jeffrey A. Brown, Advisor Between 1984 and 1991, heavy metal became one of the most publicly popular and commercially successful rock music subgenres. The focus of this dissertation is to explore the following research questions: How did the subculture of heavy metal music between 1984 and 1991 evolve and what meanings can be derived from this ongoing process? How did the contextual circumstances surrounding heavy metal music during this period impact the performative choices exhibited by artists, and from a position of retrospection, what lasting significance does this particular era of heavy metal merit today? A textual analysis of metal- related materials fostered the development of themes relating to the selective choices made and performances enacted by metal artists. These themes were then considered in terms of gender, sexuality, race, and age constructions as well as the ongoing negotiations of the metal artist within multiple performative realms. Occurring at the juncture of art and commerce, heavy metal music is a purposeful construction. Metal musicians made performative choices for serving particular aims, be it fame, wealth, or art. These same individuals worked within a greater system of influence. Metal bands were the contracted employees of record labels whose own corporate aims needed to be recognized. -
Milk and Dairy Beef Drug Residue Prevention
Milk and Dairy Beef Drug Residue Prevention Producer Manual of Best Management Practices 2014 Connecting Cows, Cooperatives, Capitol Hill, and Consumers www.nmpf.org email: [email protected] National Milk Producers Federation (“NMPF”) does not endorse any of the veterinary drugs or tests identified on the lists in this manual. The lists of veterinary drugs and tests are provided only to inform producers what products may be available, and the producer is responsible for determining whether to use any of the veterinary drugs or tests. All information regarding the veterinary drugs or tests was obtained from the products’ manufacturers or sponsors, and NMPF has made no further attempt to validate or corroborate any of that information. NMPF urges producers to consult with their veterinarians before using any veterinary drug or test, including any of the products identified on the lists in this manual. In the event that there might be any injury, damage, loss or penalty that results from the use of these products, the manufacturer of the product, or the producer using the product, shall be responsible. NMPF is not responsible for, and shall have no liability for, any injury, damage, loss or penalty. ©2014 National Milk Producers Federation Cover photo courtesy of DMI FOREWORD The goal of our nation’s dairy farmers is to produce the best tasting and most wholesome milk possible. Our consumers demand the best from us and we meet the needs of our consumers every day. Day in and day out, we provide the best in animal husbandry and animal care practices for our animals. -
Download Thesis
This electronic thesis or dissertation has been downloaded from the King’s Research Portal at https://kclpure.kcl.ac.uk/portal/ New approaches to radionuclide imaging of cancer with gallium-68 Imberti, Cinzia Awarding institution: King's College London The copyright of this thesis rests with the author and no quotation from it or information derived from it may be published without proper acknowledgement. END USER LICENCE AGREEMENT Unless another licence is stated on the immediately following page this work is licensed under a Creative Commons Attribution-NonCommercial-NoDerivatives 4.0 International licence. https://creativecommons.org/licenses/by-nc-nd/4.0/ You are free to copy, distribute and transmit the work Under the following conditions: Attribution: You must attribute the work in the manner specified by the author (but not in any way that suggests that they endorse you or your use of the work). Non Commercial: You may not use this work for commercial purposes. No Derivative Works - You may not alter, transform, or build upon this work. Any of these conditions can be waived if you receive permission from the author. Your fair dealings and other rights are in no way affected by the above. Take down policy If you believe that this document breaches copyright please contact [email protected] providing details, and we will remove access to the work immediately and investigate your claim. Download date: 05. Oct. 2021 New approaches to radionuclide imaging of cancer with gallium-68 A thesis submitted by Cinzia Imberti In partial fulfilment of the requirements for the degree of Doctor of Philosophy University of London 2018 School of Biomedical Engineering and Imaging Sciences King’s College London 1 Abstract The positron emitting isotope 68Ga is gaining increasing interest in the radionuclide imaging community, due to its favourable decay properties and availability from a generator. -
Multi-Residual Quantitative Analytical Method for Antibiotics in Sea Food by LC/MS/MS
PO-CON1742E Multi-residual quantitative analytical method for antibiotics in sea food by LC/MS/MS ASMS 2017 TP 198 Anant Lohar, Shailendra Rane, Ashutosh Shelar, Shailesh Damale, Rashi Kochhar, Purushottam Sutar, Deepti Bhandarkar, Ajit Datar, Pratap Rasam and Jitendra Kelkar Shimadzu Analytical (India) Pvt. Ltd., 1 A/B, Rushabh Chambers, Makwana Road, Marol, Andheri (E), Mumbai-400059, Maharashtra, India. Multi-residual quantitative analytical method for antibiotics in sea food by LC/MS/MS Introduction Antibiotics are widely used in agriculture as growth LC/MS/MS method has been developed for quantitation of enhancers, disease treatment and control in animal feeding multi-residual antibiotics (Table 1) from sea food sample operations. Concerns for increased antibiotic resistance of using LCMS-8040, a triple quadrupole mass spectrometer microorganisms have prompted research into the from Shimadzu Corporation, Japan. Simultaneous analysis environmental occurrence of these compounds. of multi-residual antibiotics often exhibit peak shape Assessment of the environmental occurrence of antibiotics distortion owing to their different chemical nature. To depends on development of sensitive and selective overcome this, autosampler pre-treatment feature was analytical methods based on new instrumental used [1]. technologies. Table 1. List of antibiotics Sr.No. Name of group Name of compound Number of compounds Flumequine, Oxolinic Acid, Ciprofloxacin, Danofloxacin, Difloxacin.HCl, 1 Fluoroquinolones 8 Enrofloxacin, Sarafloxacin HCl Trihydrate, -
(OTC) Antibiotics in the European Union and Norway, 2012
Perspective Analysis of licensed over-the-counter (OTC) antibiotics in the European Union and Norway, 2012 L Both 1 , R Botgros 2 , M Cavaleri 2 1. Public Health England (PHE), London, United Kingdom 2. Anti-infectives and Vaccines Office, European Medicines Agency (EMA), London, United Kingdom Correspondence: Marco Cavaleri ([email protected]) Citation style for this article: Both L, Botgros R, Cavaleri M. Analysis of licensed over-the-counter (OTC) antibiotics in the European Union and Norway, 2012. Euro Surveill. 2015;20(34):pii=30002. DOI: http://dx.doi.org/10.2807/1560-7917.ES.2015.20.34.30002 Article submitted on 16 September 2014 / accepted on 09 February 2015 / published on 27 August 2015 Antimicrobial resistance is recognised as a growing throughout the EU; however, there are still consider- problem that seriously threatens public health and able differences in Europe due to the different health- requires prompt action. Concerns have therefore been care structures and policies (including the extent of raised about the potential harmful effects of making pharmacist supervision for OTC medicines), reimburse- antibiotics available without prescription. Because of ment policies, and cultural differences of each Member the very serious concerns regarding further spread of State. Therefore, the availability of OTC medicines var- resistance, the over-the-counter (OTC) availability of ies in the EU and products sold as POM in certain coun- antibiotics was analysed here. Topical and systemic tries can be obtained as OTC medicines in others. OTC antibiotics and their indications were determined across 26 European Union (EU) countries and Norway As risk minimisation is an important criterion for some by means of a European survey. -
Sulfonamides and Sulfonamide Combinations*
Sulfonamides and Sulfonamide Combinations* Overview Due to low cost and relative efficacy against many common bacterial infections, sulfonamides and sulfonamide combinations with diaminopyrimidines are some of the most common antibacterial agents utilized in veterinary medicine. The sulfonamides are derived from sulfanilamide. These chemicals are structural analogues of ρ-aminobenzoic acid (PABA). All sulfonamides are characterized by the same chemical nucleus. Functional groups are added to the amino group or substitutions made on the amino group to facilitate varying chemical, physical and pharmacologic properties and antibacterial spectra. Most sulfonamides are too alkaline for routine parenteral use. Therefore the drug is most commonly administered orally except in life threatening systemic infections. However, sulfonamide preparations can be administered orally, intramuscularly, intravenously, intraperitoneally, intrauterally and topically. Sulfonamides are effective against Gram-positive and Gram-negative bacteria. Some protozoa, such as coccidians, Toxoplasma species and plasmodia, are generally sensitive. Chlamydia, Nocardia and Actinomyces species are also sensitive. Veterinary diseases commonly treated by sulfonamides are actinobacillosis, coccidioidosis, mastitis, metritis, colibacillosis, pododermatitis, polyarthritis, respiratory infections and toxo- plasmosis. Strains of rickettsiae, Pseudomonas, Klebsiella, Proteus, Clostridium and Leptospira species are often highly resistant. Sulfonamides are bacteriostatic antimicrobials -
A Rapid Multi-Residue Method for the Determination of Sulfonamide And
A RAPID MULTIRESIDUE METHOD FOR THE DETERMINATION OF SULFONAMIDE AND ß-LACTAM RESIDUES IN BOVINE MILK Gordon Kearney NOTE Waters Corporation, Manchester, UK Introduction Method Various sulfonamide and ß-lactam antibacterial Three recovery samples at 4 ppb and three at compounds may be used to treat disease in lactating 40 ppb were prepared. Matrix matched external dairy cattle and it is therefore necessary to monitor calibration standards were prepared at 0, 1, 10, 20, milk for the presence of residues of these drugs. In 50 and 100 ppb. Since 1 mL milk is equivalent to the European Union, maximum residue level (MRL) 0.5 mL final extract, a concentration of 1 ppb residue values range from 100 ppb, as a total concentration in milk is equivalent to a concentration of 2 ng/mL in of all sulfonamides, to 4 ppb for each of the penicillin the final extract. compounds amoxillin and ampicillin[1]. In the USA, Extraction the tolerance levels are 10 ppb for both these penicillin compounds, but the use of most sulfonamides 1 mL aliquots of pasteurized, homogenized cows [2] Application is prohibited in cattle used for milk production . milk, containing 4% fat, were transferred to 2 mL polypropylene sample tubes. Recovery samples Multiresidue analyses are increasingly gaining were spiked, agitated and left to equilibrate for acceptance for the determination of residues in 30 minutes. In order to separate the lipid from the foodstuffs; methods have recently been published for aqueous portion, the tubes were centrifuged at the monitoring of over 150 pesticide compounds in 13,000 rpm for 10 minutes. -
Third ESVAC Report
Sales of veterinary antimicrobial agents in 25 EU/EEA countries in 2011 Third ESVAC report An agency of the European Union The mission of the European Medicines Agency is to foster scientific excellence in the evaluation and supervision of medicines, for the benefit of public and animal health. Legal role Guiding principles The European Medicines Agency is the European Union • We are strongly committed to public and animal (EU) body responsible for coordinating the existing health. scientific resources put at its disposal by Member States • We make independent recommendations based on for the evaluation, supervision and pharmacovigilance scientific evidence, using state-of-the-art knowledge of medicinal products. and expertise in our field. • We support research and innovation to stimulate the The Agency provides the Member States and the development of better medicines. institutions of the EU the best-possible scientific advice on any question relating to the evaluation of the quality, • We value the contribution of our partners and stake- safety and efficacy of medicinal products for human or holders to our work. veterinary use referred to it in accordance with the • We assure continual improvement of our processes provisions of EU legislation relating to medicinal prod- and procedures, in accordance with recognised quality ucts. standards. • We adhere to high standards of professional and Principal activities personal integrity. Working with the Member States and the European • We communicate in an open, transparent manner Commission as partners in a European medicines with all of our partners, stakeholders and colleagues. network, the European Medicines Agency: • We promote the well-being, motivation and ongoing professional development of every member of the • provides independent, science-based recommenda- Agency. -
Danmap 2006.Pmd
DANMAP 2006 DANMAP 2006 DANMAP 2006 - Use of antimicrobial agents and occurrence of antimicrobial resistance in bacteria from food animals, foods and humans in Denmark Statens Serum Institut Danish Veterinary and Food Administration Danish Medicines Agency National Veterinary Institute, Technical University of Denmark National Food Institute, Technical University of Denmark Editors: Hanne-Dorthe Emborg Danish Zoonosis Centre National Food Institute, Technical University of Denmark Mørkhøj Bygade 19 Contents DK - 2860 Søborg Anette M. Hammerum National Center for Antimicrobials and Contributors to the 2006 Infection Control DANMAP Report 4 Statens Serum Institut Artillerivej 5 DK - 2300 Copenhagen Introduction 6 DANMAP board: National Food Institute, Acknowledgements 6 Technical University of Denmark: Ole E. Heuer Frank Aarestrup List of abbreviations 7 National Veterinary Institute, Tecnical University of Denmark: Sammendrag 9 Flemming Bager Danish Veterinary and Food Administration: Summary 12 Justin C. Ajufo Annette Cleveland Nielsen Statens Serum Institut: Demographic data 15 Dominique L. Monnet Niels Frimodt-Møller Anette M. Hammerum Antimicrobial consumption 17 Danish Medicines Agency: Consumption in animals 17 Jan Poulsen Consumption in humans 24 Layout: Susanne Carlsson Danish Zoonosis Centre Resistance in zoonotic bacteria 33 Printing: Schultz Grafisk A/S DANMAP 2006 - September 2007 Salmonella 33 ISSN 1600-2032 Campylobacter 43 Text and tables may be cited and reprinted only with reference to this report. Resistance in indicator bacteria 47 Reprints can be ordered from: Enterococci 47 National Food Institute Escherichia coli 58 Danish Zoonosis Centre Tecnical University of Denmark Mørkhøj Bygade 19 DK - 2860 Søborg Resistance in bacteria from Phone: +45 7234 - 7084 diagnostic submissions 65 Fax: +45 7234 - 7028 E. -
Investigation of Novel Nanoparticles of Gallium Ferricyanide and Gallium Lawsonate As Potential Anticancer Agents, and Nanoparti
Investigation of Novel Nanoparticles of Gallium Ferricyanide and Gallium Lawsonate as Potential Anticancer Agents, and Nanoparticles of Novel Bismuth Tetrathiotungstate as Promising CT Contrast Agent A Thesis submitted to Kent State University In partial fulfillment of the requirements for the degree of Master of Science Liu Yang August 2014 Thesis written by Liu Yang B.S. Kent State University, 2013 M.S. Kent State University, 2014 Approved by ___________________________________, Advisor, Committee member Dr. Songping Huang ___________________________________, Committee member Dr. Scott Bunge ___________________________________, Committee member Dr. Mietek Jaroniec Accepted by ___________________________________, Chair, Department of Chemistry Dr. Michael Tubergen ___________________________________, Dean, College of Arts and Sciences Dr. James L. Blank ii Table of Contents List of Figures..…………………………………………………………………........vii Acknowledgements ……………………………………………………………….….xi Chapter 1: Summary, Materials and Methods …..……………………………………1 1.1 Materials ………………………………………………………………….3 1.1.1 carboxymethyl reduced polysaccharide (CMRD) preparation….3 1.2 Methods …………………………………………………………………4 1.2.1 Atomic absorption spectroscopy (AA) …………………………4 1.2.2 Acid base treating method ……………………………………...4 1.2.3 Cell viability study ……………………………………………...5 i) MTT assay…………………………………………………..5 ii) Trypan blue assay ………………………………………….6 1.2.4 Dialysis …………………………………………………………6 1.2.5 Elementary analysis …………………………………………….7 1.2.6 Lyophilization …………………………………………………..7 iii 1.2.7 -
Accept Albums Metal Blast from the Past DVD / CD
Subba-Cultcha Page 1 of 3 *HOME *CONTACT To view all DVD reviews click h PODCAST FESTIVALS Homepage Accept Albums Metal Blast From The Past DVD / CD Singles & EPs Breeze Music / Drakkar Entertainment / BMG Live Features “The ultimate DVD / CD package from the epitome of Germ metal…” Demos Homepage Film In 1985, I was introduced to a band that would change my life. They made me rethink everything I knew about metal, made me realise that whilst metal may have been born DVD in the UK, and may have become the staple diet of teenage Americans, it’s real home Games and future lay in Germany. The band was Accept, and the record that changed Books everything for me went by the name of “Metal Heart”. It was, and is, everything that I thought metal should always be - overblown and dramatic, crammed full of incredible songs played by some of the greatest metal musicians that Satan ever created, and th voice….Damn, Udo Dirkschneider still has the BEST voice in metal. “Metal Heart” was and is, perfect, in every sense of the word. Thus began my continuous obsession with both German metal and to a lesser extent, Accept, and twenty one years later, it still Homepage consumes me, fills my waking thoughts and drives me ever onwards in my quest to fin Forum a metal record equal to “Metal Heart”… Competiton Subba -games Enough of me though, and on to “Metal Blast From The Past”…It’s like handing a fix t a addict entering withdrawal, presenting fresh virgins to Genghis Khan and handing a oil filed to George Dubya Bush. -
ACCEPT Release Date Pre-Order Start Symphonic Terror - Live at Wacken 2017 Uu 23/11/2018 Uu 21/09/2018 Territory: World
New Release Information uu November ACCEPT Release Date Pre-Order Start Symphonic Terror - Live At Wacken 2017 uu 23/11/2018 uu 21/09/2018 Territory: World uu 05.10. digital 2-track single Balls To The Wall (live) uu a dvertising in many important music magazines SEP/OCT/NOV 2018 uu album reviews, interviews in all important Metal magazines in Europe’s SEP/OCT/NOV 2018 issues uu song placements in European magazine compilations uu spotify playlists in all European territories uu Facebook, YouTube, Twitter, Google+ organic promotion uu Facebook ads and promoted posts + Google ads in both the search and display networks, bing ads and gmail ads uu Banner advertising on more than 60 most important Metal & Rock websites all over Europe uu additional booked ads on Metal Hammer Germany and UK, and in the Fixion network (mainly Blabbermouth) uu video and pre-roll ads on You tube uu ad campaigns on iPhones for iTunes and Google Play for Androids uu banners, featured items at the shop, header images and a background on nuclearblast.de and nuclearblast.com uu features and banners in newsletters, as well as special mailings to targeted audiences in support of the release Price Code: DVD01 Price Code: DVD02 Price Code: CD15 Price Code: CD02 Price Code: CD15 NB 4499-0 BluRay+2CD-Digi NB 4499-2 DVD+2CD-Digi NB 4499-4 Earbook NB 4573-0 2CD-Digi NB 4499-1 3LP BOX BluRay + DVD + 2CD black incl. booklet and poster uu Tracklists: Blu-ray / DVD (Blu-ray+2CD, DVD+2CD, Earbook) CD1 (Blu-ray+2CD, DVD+2CD, 2CD-Digi, Earbook) 3LP (33 rpm) Part 1: Accept 01.