Ascidian Toxins with Potential for Drug Development
Total Page:16
File Type:pdf, Size:1020Kb
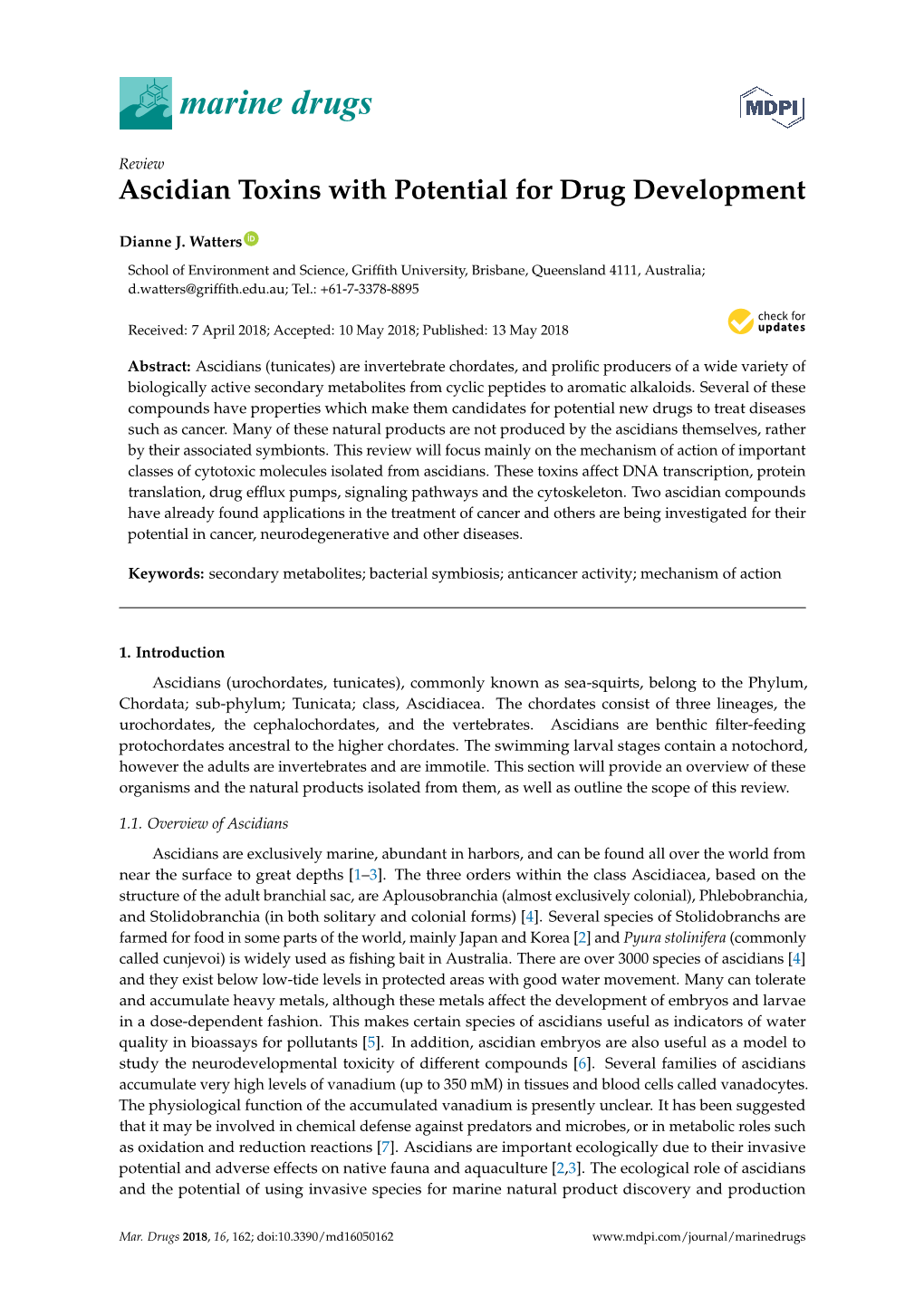
Load more
Recommended publications
-
Natural Products Diversity of Marine Ascidians (Tunicates; Ascidiacea) and Successful Drugs in Clinical Development
Nat. Prod. Bioprospect. DOI 10.1007/s13659-016-0115-5 REVIEW Natural Products Diversity of Marine Ascidians (Tunicates; Ascidiacea) and Successful Drugs in Clinical Development Satheesh Kumar Palanisamy . N. M. Rajendran . Angela Marino Received: 19 November 2016 / Accepted: 14 December 2016 Ó The Author(s) 2017. This article is published with open access at Springerlink.com Abstract This present study reviewed the chemical diversity of marine ascidians and their pharmacological applications, challenges and recent developments in marine drug discovery reported during 1994–2014, highlighting the structural activity of compounds produced by these specimens. Till date only 5% of living ascidian species were studied from\3000 species, this study represented from family didemnidae (32%), polyclinidae (22%), styelidae and polycitoridae (11–12%) exhibiting the highest number of promising MNPs. Close to 580 compound structures are here discussed in terms of their occurrence, structural type and reported biological activity. Anti-cancer drugs are the main area of interest in the screening of MNPs from ascidians (64%), followed by anti-malarial (6%) and remaining others. FDA approved ascidian compounds mechanism of action along with other compounds status of clinical trials (phase 1 to phase 3) are discussed here in. This review highlights recent developments in the area of natural products chemistry and biotechnological approaches are emphasized. Keywords Cancer Á Cytotoxicity Á Diversity Á Metabolites Á Pharmacology 1 Introduction from marine invertebrates, especially sponges, ascidians, bryozoans and molluscs in which some of them are The study of marine natural products (MNPs) is becoming approved by FDA and currently utilized in clinical trials ever more sophisticated and an increasingly collaborative [1]. -
Redalyc.Keys for the Identification of Families and Genera of Atlantic
Biota Neotropica ISSN: 1676-0611 [email protected] Instituto Virtual da Biodiversidade Brasil Moreira da Rocha, Rosana; Bastos Zanata, Thais; Moreno, Tatiane Regina Keys for the identification of families and genera of Atlantic shallow water ascidians Biota Neotropica, vol. 12, núm. 1, enero-marzo, 2012, pp. 1-35 Instituto Virtual da Biodiversidade Campinas, Brasil Available in: http://www.redalyc.org/articulo.oa?id=199123750022 How to cite Complete issue Scientific Information System More information about this article Network of Scientific Journals from Latin America, the Caribbean, Spain and Portugal Journal's homepage in redalyc.org Non-profit academic project, developed under the open access initiative Keys for the identification of families and genera of Atlantic shallow water ascidians Rocha, R.M. et al. Biota Neotrop. 2012, 12(1): 000-000. On line version of this paper is available from: http://www.biotaneotropica.org.br/v12n1/en/abstract?identification-key+bn01712012012 A versão on-line completa deste artigo está disponível em: http://www.biotaneotropica.org.br/v12n1/pt/abstract?identification-key+bn01712012012 Received/ Recebido em 16/07/2011 - Revised/ Versão reformulada recebida em 13/03/2012 - Accepted/ Publicado em 14/03/2012 ISSN 1676-0603 (on-line) Biota Neotropica is an electronic, peer-reviewed journal edited by the Program BIOTA/FAPESP: The Virtual Institute of Biodiversity. This journal’s aim is to disseminate the results of original research work, associated or not to the program, concerned with characterization, conservation and sustainable use of biodiversity within the Neotropical region. Biota Neotropica é uma revista do Programa BIOTA/FAPESP - O Instituto Virtual da Biodiversidade, que publica resultados de pesquisa original, vinculada ou não ao programa, que abordem a temática caracterização, conservação e uso sustentável da biodiversidade na região Neotropical. -
Atlas De La Faune Marine Invertébrée Du Golfe Normano-Breton. Volume
350 0 010 340 020 030 330 Atlas de la faune 040 320 marine invertébrée du golfe Normano-Breton 050 030 310 330 Volume 7 060 300 060 070 290 300 080 280 090 090 270 270 260 100 250 120 110 240 240 120 150 230 210 130 180 220 Bibliographie, glossaire & index 140 210 150 200 160 190 180 170 Collection Philippe Dautzenberg Philippe Dautzenberg (1849- 1935) est un conchyliologiste belge qui a constitué une collection de 4,5 millions de spécimens de mollusques à coquille de plusieurs régions du monde. Cette collection est conservée au Muséum des sciences naturelles à Bruxelles. Le petit meuble à tiroirs illustré ici est une modeste partie de cette très vaste collection ; il appartient au Muséum national d’Histoire naturelle et est conservé à la Station marine de Dinard. Il regroupe des bivalves et gastéropodes du golfe Normano-Breton essentiellement prélevés au début du XXe siècle et soigneusement référencés. Atlas de la faune marine invertébrée du golfe Normano-Breton Volume 7 Bibliographie, Glossaire & Index Patrick Le Mao, Laurent Godet, Jérôme Fournier, Nicolas Desroy, Franck Gentil, Éric Thiébaut Cartographie : Laurent Pourinet Avec la contribution de : Louis Cabioch, Christian Retière, Paul Chambers © Éditions de la Station biologique de Roscoff ISBN : 9782951802995 Mise en page : Nicole Guyard Dépôt légal : 4ème trimestre 2019 Achevé d’imprimé sur les presses de l’Imprimerie de Bretagne 29600 Morlaix L’édition de cet ouvrage a bénéficié du soutien financier des DREAL Bretagne et Normandie Les auteurs Patrick LE MAO Chercheur à l’Ifremer -
Download (4MB)
POPULATION DYNAMICS OF A NON-INDIGENOUS COLONIAL ASCIDIAN TUNICATE IN A SUBARCTIC HARBOUR by ©KEVIN C. K. MA A thesis submitted to the School of Graduate Studies in partial fulfillment of the requirements for the degree of MASTER OF SCIENCE DEPARTMENT OF BIOLOGY, FACULTY OF SCIENCE MEMORIAL UNIVERSITY OF NEWFOUNDLAND December 2012 ST. JOHN'S NEWFOUNDLAND AND LABRADOR CANADA ABSTRACT Botryllus schlosseri (Subphylum Tunicata: Class Ascidiacea) is a non indigenous asci dian species of global and national interest, which has extensive populations along the south coast of insular Newfoundland. Economically, this species has been of concern to industry, management, and policymakers because non-indigenous ascidian species have been a severe and costly nuisance for bivalve aquaculture. Ecologically, the presence of this temperate-adapted species in Newfoundland represents an expansion of its global range into subarctic waters. Thus, I aimed to describe the population dynamics of B. schlosseri in Arnold's Cove, Placentia Bay, Newfoundland and Labrador, Canada, by determining the temporal and spatial patterns of recruitment and the seasonal cycle of colony abundance. In addition, I aimed to compile a checklist of extant indigenous and non-indigenous ascidian species of eastern Canada with an emphasis on species from Newfoundland and Labrador. Artificial plates were used to determine recruitment rates among three sites, depths (1.0, 2.5, and 4.0 m from the water surface), and substrate types (aluminum, PVC, and wood in 2010; only PVC in 2011), in Arnold's Cove. Concurrently, density and cover of colonies were determined from the analysis of high-definition video surveys of a belt transect of wharf pilings. -
Composition and Application Potentials of Scandinavian Tunicates
Master Thesis Report KTH Chemical Science and Engineering Composition and Application Potentials of Scandinavian Tunicates Masoumeh Hassanzadeh Supervisor: Docent Jiebing Li Examiner: Professor Michael E. Lindström 20 October 2011 i Abstract Marine ecosystems can be a promising reservoir of various kinds of chemical components, applicable as pharmaceutical materials, food, cosmetics, nutraceuticals, and others for different industry. As an example, Tunicates, a group of marine animals, have been attracted a lot of attention in medical application, food market, water pollution issues, and Cellulose nanomaterial production due to their consisting of chemical compounds such as cellulose, amino-sugars, and proteins or protein-polysaccharide complexes e.g. collagen, glycosaminoglycan, chitin, scleroprotein, iodine-binding proteins, and elastin. In this project, two dominant species of Scandinavian Tunicates, i.e. Ciona intestinalis and Clavelina lepadiformis, harvested from Norwegian ocean have been classified according to body sizes, depths from the ocean surface, ages and species, and separated physically into outer layer and internal organs, followed by measurements of sugar composition, oil content, and protein content. Application potentials have been investigated by trials for production of pure crystalline cellulose, bioethanol, and biodiesel, and by analysis of amino acid composition of the samples. The cellulose percentage and cellulose yield for the chemically pure cellulose obtained, is around 96% and 54% respectively, and the protein content is decreased step by step by the acid, alkali, and bleaching process applied. Bioethanol can be obtained by fermentation of tunicate hydrolysate with strains A and C which are derived from Saccharomyces cerevisiae. The biodiesel yield of tunicate samples is around 4-6% as an average. -
Title CONTRIBUTIONS to JAPANESE ASCIDIAN FAUNA. XX
CONTRIBUTIONS TO JAPANESE ASCIDIAN FAUNA. XX. -THE OUTLINE OF JAPANESE ASCIDIAN FAUNA Title AS COMPARED WITH THAT OF THE PACIFIC COASTS OF NORTH AMERICA- Author(s) Tokioka, Takasi PUBLICATIONS OF THE SETO MARINE BIOLOGICAL Citation LABORATORY (1963), 11(1): 131-156 Issue Date 1963-07-20 URL http://hdl.handle.net/2433/175319 Right Type Departmental Bulletin Paper Textversion publisher Kyoto University CONTRIBUTIONS TO JAPANESE ASCIDIAN FAUNA. XX. THE OUTLINE OF JAPANESE ASCIDIAN FAUNA AS COMPARED WITH 1 2 THAT OF THE PACIFIC COASTS OF NORTH AMERICA. • ) T AKASI TOKIOKA Seto Marine Biological Laboratory L The outline of the ascidian fauna of the Japanese waters The review of the ascidian fauna of the Japanese waters was first made by HARTMEYER* who referred in his paper of 1906 to 43 species including a number of those that were newly established or recorded by himself form the Japanese waters. About thirty years later, the late Prof. Asajir6 0KA** made the second review (1935) on Japanese ascidian fauna as he closed his studies on ascidians for about forty years. He mentioned that there were 106 ascidian species known from the Japanese waters and that of these species endemic ones were the most abundant, though 8 arctic and 6 tropical species and 2 cosmopolitans were included. Further he divided the endemic species into two groups, the northern and southern groups; the former comprised the species occurring in the waters surrounding Hokkaido Island and the northern half of Honsyfi Island, while the latter consisted of ones living in the waters along the coasts of Kyfisyfi and Sikoku Islands and the southern half of Honsyfi Island. -
Phylogenetic Analysis of Phenotypic Characters of Tunicata Supports Basal Appendicularia and Monophyletic Ascidiacea
Cladistics Cladistics 36 (2020) 259–300 10.1111/cla.12405 Phylogenetic analysis of phenotypic characters of Tunicata supports basal Appendicularia and monophyletic Ascidiacea Katrin Brauna, Fanny Leubnerb and Thomas Stachc,* aVergleichende Zoologie, Institut fur€ Biologie, Humboldt-Universitat€ zu Berlin, Philippstrasse 13, Haus 2, 10115 Berlin, Germany; bAnimal Evolution and Biodiversity, J-F-Blumenbach Institute for Zoology & Anthropology, Georg-August-University Gottingen,€ Untere Karspule€ 2, 37073 Gottingen,€ Germany; cMolekulare Parasitologie, Institut fur€ Biologie, Humboldt-Universitat€ zu Berlin, Philippstrasse 13, Haus 14, 10115 Berlin, Germany Abstract With approximately 3000 marine species, Tunicata represents the most disparate subtaxon of Chordata. Molecular phyloge- netic studies support Tunicata as sister taxon to Craniota, rendering it pivotal to understanding craniate evolution. Although successively more molecular data have become available to resolve internal tunicate phylogenetic relationships, phenotypic data have not been utilized consistently. Herein these shortcomings are addressed by cladistically analyzing 117 phenotypic characters for 49 tunicate species comprising all higher tunicate taxa, and five craniate and cephalochordate outgroup species. In addition, a combined analysis of the phenotypic characters with 18S rDNA-sequence data is performed in 32 OTUs. The analysis of the combined data is congruent with published molecular analyses. Successively up-weighting phenotypic characters indicates that phenotypic data contribute disproportionally more to the resulting phylogenetic hypothesis. The strict consensus tree from the analysis of the phenotypic characters as well as the single most parsimonious tree found in the analysis of the combined dataset recover monophyletic Appendicularia as sister taxon to the remaining tunicate taxa. Thus, both datasets support the hypothesis that the last common ancestor of Tunicata was free-living and that ascidian sessility is a derived trait within Tunicata. -
13 Acorn-Worms and Sea Squirts
1 13 ACORN-WORMS AND SEA SQUIRTS PHYLUM CHORDATA: SUBPHYLUM UROCHORDATA (= TUNICATA) Class Ascidiacea The tunicate body is contained within a test, or tunic, secreted by the underlying mantle. This test may be thin, thick, clear, opaque, clean, or covered with sand or living organisms. Tunicates are represented on most rocky shores by the ascidians, which are sessile and either colonial or unitary. Some are indefinite in shape, and so hidden within their tunics that they superficially resemble sponges or some of the more fleshy bryozoans or cnidarians (e.g. Alcyonidium or Alcyonium). When cut open, however, they display a more complicated internal organization (Fig. 13.2), particularly a filter-feeding pharynx, or branchial sac, in which mucus is secreted by the ventral endostyle. The branchial sac contains perforations, the stigmata, in transverse rows. Transverse blood vessels pass between adjacent rows. In some genera the stigmata are arranged in spiral groups. The feeding current enters the test via an oral (or branchial) opening or siphon, and passes into the pharynx through a ring of oral tentacles. Flow is generated by cilia, passing through the stigmata into the surrounding atrium. From there the water passes to the exterior via a dorsal atrial (excurrent) siphon. Positive pressure builds up inside the atrium, which becomes distended with water. On disturbance, contraction of mantle muscles forces out jets of water through the oral and atrial sphincters, hence the term ‘sea squirts’ popularly applied to larger ascidians. Unitary ascidians may be 1 cm long or more, and the largest British forms reach about 15 cm. -
10. Svalgsträngsdjur Till Ryggradsdjur
svag ringfåra nära bakkanten. Gonaderna börjar uppträda i HEMICHORDATA W. Bateson, 1885 höjd med de mittre gälporerna. På metasomet kan små röda {hemikårdáta} (5–7 gen., 7–11 sp.) fläckar förekomma. S. pygmaeus blir blott ≤3 cm lång, har [Gr. hemi = halv + phylum Chordata : (se nedan)] 9–22 gälporpar, det ≈4 ggr längre än breda prosomet saknar Bilateralsymmetriska, osegmenterade, solitära el. koloni- längsfåror och är täckt av små vårtor. Dess färg är mjölkvit bildande, bentiska marina djur, med kropp bestående av 3 till ljusgul, medan kragen är ngt mörkare gul el. gulbrun. avsnitt, pro-, mesooch metasom (proboscis, krage och Kragen är bredare än lång med en ringfåra nära bakänden. bakkropp). Förutom de båda nedanstående klasserna plägar Gonader börjar uppträda bakom gälregionen. En 4:e, en oceanisk, geleartad, sfärisk, ≈1 cm Ø, ciliebandförsedd rödaktig art (släktets typart) med mörkare röd krage, S. larv från Biscaya & Bermudas, kallad Planctosphaera mereschowskii (Nicolas Wagner, 1885) [Konstantin Sergeje- pelagica Spengel, 1932 ges status av egen klass, Plancto- wicz Mereschkowski, 1854–1921, rysk protistolog, som sphaeroidea Van der Horst, 1936. Totalt är 92 (106?) tidigare hade honorerat Wagner med släktnamnet Wagnerella] recenta arter kända. är känd från Arktis (närmast från Vita havet och märkligt nog påstådd vara allmän i Adriatiska Havet, ehuru den senare ENTEROPNEUSTA Gegenbaur, 1870 populationen möjl. kan vara felbestämd). Den tycks ej nå en {enteråpnévsta} "Ollonmaskar" (4–6 gen., 6–10 sp.) längd av 1 dm & kännetecknas av att prosomet har en djup [Gr. enteron = tarm, inälva + Gr. pneusticos = för andning < Gr. dorsal längsfåra korsad av finare tvärfåror, kragen är lika pneumon = lunga] lång som bred & de ≈50 gälpor-paren börjar medio- Solitära, rörliga, externt rikt cilierade, skildkönade, branchialt. -
Secondary Metabolites from Polar Organisms
marine drugs Review Secondary Metabolites from Polar Organisms Yuan Tian 1,*, Yan-Ling Li 1 and Feng-Chun Zhao 2 1 College of Life Science, Taishan Medical University, Taian 271016, Shandong, China; [email protected] 2 College of Life Science, Shandong Agricultural University, Taian 271018, Shandong, China; [email protected] * Correspondence: [email protected]; Tel.: +86-358-623-5778 Academic Editor: Orazio Taglialatela-Scafati Received: 7 January 2017; Accepted: 29 January 2017; Published: 23 February 2017 Abstract: Polar organisms have been found to develop unique defences against the extreme environment environment, leading to the biosynthesis of novel molecules with diverse bioactivities. This review covers the 219 novel natural products described since 2001, from the Arctic and the Antarctic microoganisms, lichen, moss and marine faunas. The structures of the new compounds and details of the source organism, along with any relevant biological activities are presented. Where reported, synthetic and biosynthetic studies on the polar metabolites have also been included. Keywords: natural products; secondary metabolism; structure elucidation; biological activity; biosynthesis; chemical synthesis 1. Introduction Organisms from special ecosystems such as the polar regions are a rich source of various chemical scaffolds and novel natural products with promising bioactivities. Polar regions, which refer to the Arctic, the Antarctic and their subregions, are remote and challenging areas on the earth. To survive under the constant influence of low temperatures, strong winds, low nutrient and high UV radiation or combinations of these factors [1], polar organisms require a diverse array of biochemical and physiological adaptations that are essential for survival. These adaptations are often accompanied by modifications to both gene regulation and metabolic pathways, increasing the possibility of finding unique functional metabolites of pharmaceutical importance. -
Haydar, Deniz
University of Groningen What is natural? Haydar, Deniz IMPORTANT NOTE: You are advised to consult the publisher's version (publisher's PDF) if you wish to cite from it. Please check the document version below. Document Version Publisher's PDF, also known as Version of record Publication date: 2010 Link to publication in University of Groningen/UMCG research database Citation for published version (APA): Haydar, D. (2010). What is natural? The scale and consequences of marine bioinvasions in the North Atlantic Ocean. s.n. Copyright Other than for strictly personal use, it is not permitted to download or to forward/distribute the text or part of it without the consent of the author(s) and/or copyright holder(s), unless the work is under an open content license (like Creative Commons). Take-down policy If you believe that this document breaches copyright please contact us providing details, and we will remove access to the work immediately and investigate your claim. Downloaded from the University of Groningen/UMCG research database (Pure): http://www.rug.nl/research/portal. For technical reasons the number of authors shown on this cover page is limited to 10 maximum. Download date: 24-09-2021 Appendix I to Chapter 4: The scale of cryptogenesis, in: Deniz Haydar (2010) What is natural? The scale and consequences of marine bioinvasions in the North Atlantic Ocean. Dissertation, University of Groningen, 184 pp. ISBN 978-90-367-4396-9 ISBN 978-90-367-4395-2 available at http://dissertations.ub.rug.nl/faculties/science/2010/ Appendix I Ascidiacea species in the North Atlantic Ocean, excluding disjunct amphi-Atlantic species (see table 4.2). -
Nuevos Datos Sobre Cuatro Especies De Ascidias De La
ANALES DE BIOLOG~A,19 (Biología Animal, 8) 1993: 19-27 SECRETARIADO DE PUBLICACIONES - UNIVERSIDAD DE MURCIA NUEVOS DATOS SOBRE CUATRO ESPECIES DE ASCIDIAS DE LA FAMILIA PoLYCLINZDAE EN LA PEN~NSULAIB~RICA Elsa Vázquez y Victoriano Urgorri * Recibido: 22 marzo 1993 Aceptado: 12 enero 1994 SUMMARY New data about distribution of four species of ascidians of thepolyclynidae family in the Iberian Peninsula New data about the distribution of four species of ascidians in the Iberian Peninsula coast are given: Aplidium glabrum (Verri1.187 1) and Synoicum pulmonaria (Ellis & Solander, 1786) are recorded for the first time in Iberian Peninsula, Aplidium punctum (Giard, 1873) is recorded for the first time in the Spanish coast and A. asperum (Drasche, 1883) for the Atlantic Iberian coasts. The tadpole lawae of A. glabrum is described for the first time and its northem distribution limit is enlarged. The synonymy between A. asperum and A. coeruleum is discussed. Key words: Aplidium, Synoicum, Polyclinidae, Ascidiaceae, description, distribution, Iberian Penin- sula. RESUMEN Se aportan nuevos datos sobre la distribución de cuatro especies de ascidias en las costas de la Penín- sula Ibérica: Aplidium glabrum (Verril, 1871) y Synoicum pulmonaria (Ellis & Solander, 1786) son nuevas citas en la Península Ibérica, Aplidium punctum (Giard, 1873) lo es en las costas españolas y A. asperum (Drasche, 1883) en las costas atlánticas ibéricas. Se describe por primera vez la lawa de A. glabrum y se amplía su límite de distribución meridional hasta nuestras costas. Se discute la sinonimia entre A. asperum y A. coeruleum. Palabras clave: Aplidium, Synoicum, Polyclinidae, Ascidiacea, descripción, distribución, Península Ibérica.