Nonlinear Magic: Multiphoton Microscopy in the Biosciences
Total Page:16
File Type:pdf, Size:1020Kb
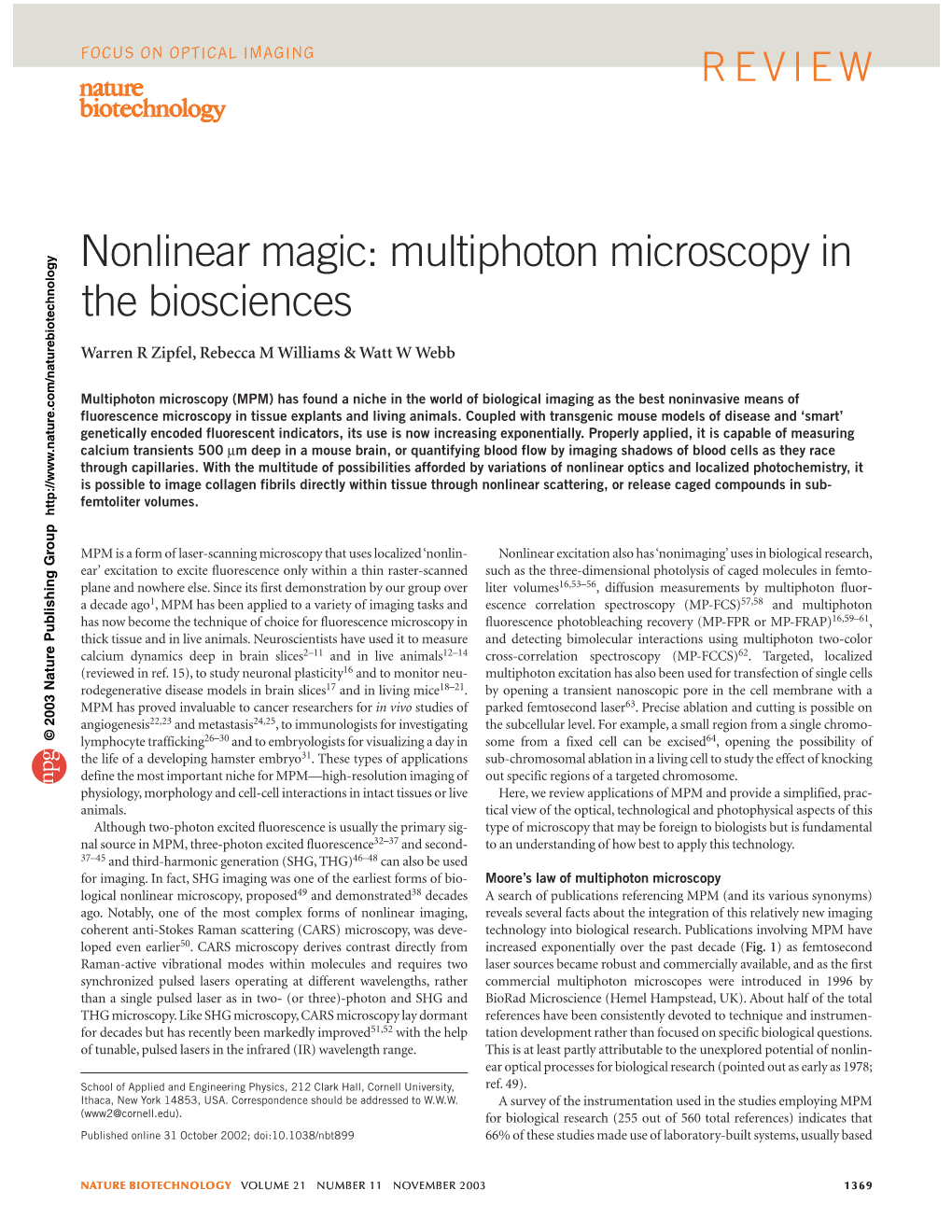
Load more
Recommended publications
-
Two-Photon Excitation, Fluorescence Microscopy, and Quantitative Measurement of Two-Photon Absorption Cross Sections
Portland State University PDXScholar Dissertations and Theses Dissertations and Theses Fall 12-1-2017 Two-Photon Excitation, Fluorescence Microscopy, and Quantitative Measurement of Two-Photon Absorption Cross Sections Fredrick Michael DeArmond Portland State University Let us know how access to this document benefits ouy . Follow this and additional works at: https://pdxscholar.library.pdx.edu/open_access_etds Part of the Physics Commons Recommended Citation DeArmond, Fredrick Michael, "Two-Photon Excitation, Fluorescence Microscopy, and Quantitative Measurement of Two-Photon Absorption Cross Sections" (2017). Dissertations and Theses. Paper 4036. 10.15760/etd.5920 This Dissertation is brought to you for free and open access. It has been accepted for inclusion in Dissertations and Theses by an authorized administrator of PDXScholar. For more information, please contact [email protected]. Two-Photon Excitation, Fluorescence Microscopy, and Quantitative Measurement of Two-Photon Absorption Cross Sections by Fredrick Michael DeArmond A dissertation submitted in partial fulfillment of the requirements for the degree of Doctor of Philosophy in Applied Physics Dissertation Committee: Erik J. Sánchez, Chair Erik Bodegom Ralf Widenhorn Robert Strongin Portland State University 2017 ABSTRACT As optical microscopy techniques continue to improve, most notably the development of super-resolution optical microscopy which garnered the Nobel Prize in Chemistry in 2014, renewed emphasis has been placed on the development and use of fluorescence microscopy techniques. Of particular note is a renewed interest in multiphoton excitation due to a number of inherent properties of the technique including simplified optical filtering, increased sample penetration, and inherently confocal operation. With this renewed interest in multiphoton fluorescence microscopy, comes increased interest in and demand for robust non-linear fluorescent markers, and characterization of the associated tool set. -
Replace This with the Actual Title Using All Caps
THREE-PHOTON MICROSCOPY AT 1700 NM FOR IN VIVO IMAGING A Dissertation Presented to the Faculty of the Graduate School of Cornell University In Partial Fulfillment of the Requirements for the Degree of Doctor of Philosophy by Nicholas Geoffrey Horton May 2015 © 2015 Nicholas Geoffrey Horton THREE-PHOTON MICROSCOPY AT 1,700 NM FOR IN VIVO IMAGING Nicholas Geoffrey Horton, Ph. D. Cornell University 2015 Multiphoton fluorescence microscopy (MPM) allows scientists to noninvasively observe structures deep within tissue. Two-photon microscopy (2PM) in the 750-1000 nm excitation region has been the standard MPM technique since its first demonstration in 1990. However, the maximum imaging depth for 2PM is limited by the signal-to-background ratio (SBR). In this dissertation, three-photon imaging at 1700 nm excitation wavelength (1700 nm 3PM) is presented. The combination of the long excitation wavelength and the higher order nonlinear excitation overcomes the SBR limitations of 2PM, enabling biological investigations to take place at greater depth within tissue. In chapter 1, tissue imaging is discussed, paying special attention to the development of laser scanning fluorescence microscopy. In chapter 2, non-invasive, high resolution, in vivo imaging of subcortical structures within an intact mouse brain using 1700 nm 3PM is presented. Vascular structures as well as red fluorescent protein (RFP)-labeled neurons within the mouse hippocampus are imaged. In chapter 3, dispersion compensation of 1700 nm 3PM is discussed. Signal generation in 3PM is proportional to the inverse-squared of the pulse width. We show that the high normal dispersion of a silicon wafer can be conveniently used to compensate the anomalous dispersion of a 1,700 nm excitation three-photon microscope. -
Wide. Fast. Deep. Recent Advances in Multi-Photon Microscopy of in Vivo Neuronal Activity
TechSights Wide. Fast. Deep. Recent Advances in Multi- Photon Microscopy of in vivo Neuronal Activity https://doi.org/10.1523/JNEUROSCI.1527-18.2019 Cite as: J. Neurosci 2019; 10.1523/JNEUROSCI.1527-18.2019 Received: 2 March 2019 Revised: 27 September 2019 Accepted: 27 September 2019 This Early Release article has been peer-reviewed and accepted, but has not been through the composition and copyediting processes. The final version may differ slightly in style or formatting and will contain links to any extended data. Alerts: Sign up at www.jneurosci.org/alerts to receive customized email alerts when the fully formatted version of this article is published. Copyright © 2019 the authors 1 Wide. Fast. Deep. Recent Advances in Multi-Photon Microscopy of in vivo Neuronal Activity. 2 Abbreviated title: Recent Advances of in vivo Multi-Photon Microscopy 3 Jérôme Lecoq1, Natalia Orlova1, Benjamin F. Grewe2,3,4 4 1 Allen Institute for Brain Science, Seattle, USA 5 2 Institute of Neuroinformatics, UZH and ETH Zurich, Switzerland 6 3 Dept. of Electrical Engineering and Information Technology, ETH Zurich, Switzerland 7 4 Faculty of Sciences, University of Zurich, Switzerland 8 9 Corresponding author: Jérôme Lecoq, [email protected] 10 Number of pages: 24 11 Number of figures: 6 12 Number of tables: 1 13 Number of words for: 14 ● abstract: 196 15 ● introduction: 474 16 ● main text: 5066 17 Conflict of interest statement: The authors declare no competing financial interests. 18 Acknowledgments: We thank Kevin Takasaki and Peter Saggau (Allen Institute for Brain Science) for providing helpful 19 comments on the manuscript; we thank Bénédicte Rossi for providing scientific illustrations. -
Three-Photon Tissue Imaging Using Moxifloxacin
www.nature.com/scientificreports OPEN Three-photon tissue imaging using moxifoxacin Seunghun Lee1, Jun Ho Lee1, Taejun Wang2, Won Hyuk Jang 2, Yeoreum Yoon1, Bumju Kim2, Yong Woong Jun 3, Myoung Joon Kim4 & Ki Hean Kim1,2 Received: 14 August 2017 Moxifoxacin is an antibiotic used in clinics and has recently been used as a clinically compatible cell- Accepted: 30 May 2018 labeling agent for two-photon (2P) imaging. Although 2P imaging with moxifoxacin labeling visualized Published: xx xx xxxx cells inside tissues using enhanced fuorescence, the imaging depth was quite limited because of the relatively short excitation wavelength (<800 nm) used. In this study, the feasibility of three-photon (3P) excitation of moxifoxacin using a longer excitation wavelength and moxifoxacin-based 3P imaging were tested to increase the imaging depth. Moxifoxacin fuorescence via 3P excitation was detected at a >1000 nm excitation wavelength. After obtaining the excitation and emission spectra of moxifoxacin, moxifoxacin-based 3P imaging was applied to ex vivo mouse bladder and ex vivo mouse small intestine tissues and compared with moxifoxacin-based 2P imaging by switching the excitation wavelength of a Ti:sapphire oscillator between near 1030 and 780 nm. Both moxifoxacin-based 2P and 3P imaging visualized cellular structures in the tissues via moxifoxacin labeling, but the image contrast was better with 3P imaging than with 2P imaging at the same imaging depths. The imaging speed and imaging depth of moxifoxacin-based 3P imaging using a Ti:sapphire oscillator were limited by insufcient excitation power. Therefore, we constructed a new system for moxifoxacin-based 3P imaging using a high-energy Yb fber laser at 1030 nm and used it for in vivo deep tissue imaging of a mouse small intestine. -
Three-Photon Fluorescence Imaging of Melanin with a Dual-Wedge Confocal Scanning System
Three-photon fluorescence imaging of melanin with a dual-wedge confocal scanning system Yair Mega1*, Joseph Kerimo1, Joseph Robinson1, Ali Vakili1 , Nicolette Johnson1,3 and Charles DiMarzio1,2 1Department of Electrical and Computer Engineering, The Bernard M. Gordon Center for Subsurface Sensing and Imaging Systems (Gordon-CenSSIS); 2Department of Mechanical and Industrial Engineering Northeastern University, 360 Huntington Ave, Boston, Massachusetts, 02115, USA; 3Department of Biomedical Engineering, University of Iowa, 1402 Seamans Center, Iowa City, Iowa, 52242, USA *Corresponding author: [email protected] ABSTRACT Confocal microscopy can be used as a practical tool in non-invasive applications in medical diagnostics and evaluation. In particular, it is being used for the early detection of skin cancer to identify pathological cellular components and, potentially, replace conventional biopsies. The detection of melanin and its spatial location and distribution plays a crucial role in the detection and evaluation of skin cancer. Our previous work has shown that the visible emission from melanin is strong and can be easily observed with a near-infrared CW laser using low power. This is due to a unique step-wise, (SW) three-photon excitation of melanin. This paper shows that the same SW, 3-photon fluorescence can also be achieved with an inexpensive, continuous-wave laser using a dual-prism scanning system. This demonstrates that the technology could be integrated into a portable confocal microscope for clinical applications. The results presented here are in agreement with images obtained with the larger and more expensive femtosecond laser system used earlier. Keywords: Confocal microscopy, Melanin, Fluorescence, Risley prism 1. INTRODUCTION Confocal microscopy, invented first in the early 1960's [1], has had many improvements and is close to becoming a preliminary diagnostic tool [2-5]. -
Advanced Optical Imaging Techniques for Neurodevelopment
CONEUR-1240; NO. OF PAGES 8 Available online at www.sciencedirect.com Advanced optical imaging techniques for neurodevelopment 1,3 2,3 2 1 Yicong Wu , Ryan Christensen , Daniel Colo´ n-Ramos and Hari Shroff Over the past decade, developmental neuroscience has been science, that will greatly enhance the accessibility of the transformed by the widespread application of confocal and nervous system to researchers. two-photon fluorescence microscopy. Even greater progress is imminent, as recent innovations in microscopy now enable Imaging deeper imaging with increased depth, speed, and spatial resolution; In the rodent brain, structures like the hippocampus and reduced phototoxicity; and in some cases without external other deep brain areas are covered by a millimeter or more fluorescent probes. We discuss these new techniques and of tissue that scatter or absorb light, rendering them emphasize their dramatic impact on neurobiology, including relatively inaccessible to conventional optical imaging. the ability to image neurons at depths exceeding 1 mm, to Scattering of the visible excitation wavelengths used in observe neurodevelopment noninvasively throughout confocal microscopy limits the penetration depth to less embryogenesis, and to visualize neuronal processes or than 100 mm. In two-photon microscopy, two longer- structures that were previously too small or too difficult to target wavelength (usually near-infrared) excitation photons with conventional microscopy. are absorbed instead of a single photon. Since this process depends on the near-simultaneous absorption of two Addresses 1 photons, it is strongly enhanced when the excitation is Section on High Resolution Optical Imaging, National Institute of Biomedical Imaging and Bioengineering, National Institutes of Health, 13 concentrated in time (achieved with a pulsed excitation South Drive, Bethesda, MD 20892, United States source) and in space (at the excitation focus). -
High-Pulse-Energy Multiphoton Imaging of Neurons and Oligodendrocytes in Deep Murine Brain with a Fiber Laser
www.nature.com/scientificreports OPEN High‑pulse‑energy multiphoton imaging of neurons and oligodendrocytes in deep murine brain with a fber laser Michael J. Redlich1,2, Brad Prall3, Edesly Canto‑Said3, Yevgeniy Busarov1, Lilit Shirinyan‑Tuka1, Arafat Meah1 & Hyungsik Lim1,2* Here we demonstrate high‑pulse‑energy multiphoton microscopy (MPM) for intravital imaging of neurons and oligodendrocytes in the murine brain. Pulses with an order of magnitude higher energy (~ 10 nJ) were employed from a ytterbium doped fber laser source at a 1‑MHz repetition rate, as compared to the standard 80‑MHz Ti:Sapphire laser. Intravital imaging was performed on mice expressing common fuorescent proteins, including green (GFP) and yellow fuorescent proteins (YFP), and TagRFPt. One ffth of the average power could be used for superior depths of MPM imaging, as compared to the Ti:Sapphire laser: A depth of ~ 860 µm was obtained by imaging the Thy1‑YFP brain in vivo with 6.5 mW, and cortical myelin as deep as 400 µm ex vivo by intrinsic third‑harmonic generation using 50 mW. The substantially higher pulse energy enables novel regimes of photophysics to be exploited for microscopic imaging. The limitation from higher order phototoxicity is also discussed. Two-photon excitation microscopy (2PM) with descanned detection has a superior depth of imaging, far exceeding that of one-photon excitation microscopy1–3. Similar gain can be attained in general for multiphoton microscopy (MPM), expanding biological investigations by light microscopy from cultured cells to fresh thick tissues. By virtue of the safety of the near-infrared (NIR) excitation4, MPM has been the primary tool for intra- vital microscopy leading to breakthrough discoveries in broad biomedical felds. -
Two-Photon-Like Microscopy with Orders-Of-Magnitude Lower Illumination Intensity Via Two-Step fluorescence
ARTICLE Received 28 Jan 2015 | Accepted 24 Jul 2015 | Published 3 Sep 2015 DOI: 10.1038/ncomms9184 OPEN Two-photon-like microscopy with orders-of-magnitude lower illumination intensity via two-step fluorescence Maria Ingaramo1,*, Andrew G. York1,*, Eric J. Andrade1, Kristin Rainey1 & George H. Patterson1 We describe two-step fluorescence microscopy, a new approach to non-linear imaging based on positive reversible photoswitchable fluorescent probes. The protein Padron approximates ideal two-step fluorescent behaviour: it equilibrates to an inactive state, converts to an active state under blue light, and blue light also excites this active state to fluoresce. Both activation and excitation are linear processes, but the total fluorescent signal is quadratic, proportional to the square of the illumination dose. Here, we use Padron’s quadratic non-linearity to demonstrate the principle of two-step microscopy, similar in principle to two-photon microscopy but with orders-of-magnitude better cross-section. As with two-photon, quadratic non-linearity from two-step fluorescence improves resolution and reduces unwanted out-of-focus excitation, and is compatible with structured illumination microscopy. We also show two-step and two-photon imaging can be combined to give quartic non- linearity, further improving imaging in challenging samples. With further improvements, two- step fluorophores could replace conventional fluorophores for many imaging applications. 1 National Institute of Biomedical Imaging and Bioengineering, National Institutes of Health, Bethesda, Maryland 20892, USA. * These authors contributed equally to this work. Correspondence and requests for materials should be addressed to A.G.Y. (email: andrew.g.york þ [email protected]). NATURE COMMUNICATIONS | 6:8184 | DOI: 10.1038/ncomms9184 | www.nature.com/naturecommunications 1 & 2015 Macmillan Publishers Limited. -
Three Photon Microscopy Brain Imaging
Thinkstock32 OPTICS & PHOTONICS NEWS NOVEMBER 2013 Brain Imaging with Multiphoton Microscopy Ke Wang, Nicholas G. Horton and Chris Xu As scientists seek to unravel the mysteries of the brain, they will need to delve deeper than ever before in order to image individual neurons and their processes. Multiphoton microscopy is a promising new technology for getting there. NOVEMBER 2013 OPTICS & PHOTONICS NEWS 33 he brain initiates everything we do and pro- Optical imaging is noninvasive and pro- cesses all that we experience. Yet the mystery vides the high spatial resolution necessary of the brain rivals that of the universe. In fact, to resolve individual neurons and neuronal we probably know more about the universe processes. However, acquiring images through than we do about our own brains, which significant depths of the brain is no easy task Tconsist of approximately 100 billion neurons since brain tissue is extremely heterogeneous, and 1 trillion support cells. resulting in strong scattering by the various Elucidating how the brain works is a grand tissue components. Optical imaging technol- challenge within science. It will undoubtedly ogy will be essential in addressing these help us to better understand neurological challenges, and it will feature prominently diseases such as Alzheimer’s and Parkinson’s. in U.S. President Obama’s recently announced Research in the last several decades has pro- BRAIN initiative (see sidebar on facing page). Multiphoton microscopy (MPM), which was demonstrated more than two decades Femtosecond lasers are usually ago, has dramatically extended the depth required for multi-photon microscopy penetration of high-resolution optical imaging. -
Proceedings of Spie
PROCEEDINGS OF SPIE SPIEDigitalLibrary.org/conference-proceedings-of-spie Super-nonlinear fluorescence microscopy for high-contrast deep tissue imaging Lu Wei, Xinxin Zhu, Zhixing Chen, Wei Min Lu Wei, Xinxin Zhu, Zhixing Chen, Wei Min, "Super-nonlinear fluorescence microscopy for high-contrast deep tissue imaging," Proc. SPIE 8948, Multiphoton Microscopy in the Biomedical Sciences XIV, 894825 (28 February 2014); doi: 10.1117/12.2038753 Event: SPIE BiOS, 2014, San Francisco, California, United States Downloaded From: https://www.spiedigitallibrary.org/conference-proceedings-of-spie on 6/8/2018 Terms of Use: https://www.spiedigitallibrary.org/terms-of-use Super-nonlinear fluorescence microscopy for high-contrast deep tissue imaging Lu Weia; Xinxin Zhua; Zhixing Chena; Wei Mina,b aDepartment of Chemistry, Columbia University, New York, NY 10027 bKavli Institute for Brain Science, Columbia University, New York, NY 10027 Abstract Two-photon excited fluorescence microscopy (TPFM) offers the highest penetration depth with subcellular resolution in light microscopy, due to its unique advantage of nonlinear excitation. However, a fundamental imaging-depth limit, accompanied by a vanishing signal-to-background contrast, still exists for TPFM when imaging deep into scattering samples. Formally, the focusing depth, at which the in-focus signal and the out-of-focus background are equal to each other, is defined as the fundamental imaging- depth limit. To go beyond this imaging-depth limit of TPFM, we report a new class of super-nonlinear fluorescence microscopy for high-contrast deep tissue imaging, including multiphoton activation and imaging (MPAI) harnessing novel photo-activatable fluorophores, stimulated emission reduced fluorescence (SERF) microscopy by adding a weak laser beam for stimulated emission, and two-photon induced focal saturation imaging with preferential depletion of ground-state fluorophores at focus. -
Adaptive Optical Microscopy for Neurobiology
Available online at www.sciencedirect.com ScienceDirect Adaptive optical microscopy for neurobiology 1 1,2 Cristina Rodrı´guez and Na Ji With the ability to correct for the aberrations introduced by objective. Additionally, the optical properties of the spec- biological specimens, adaptive optics — a method originally imen and the immersion medium need to be matched. developed for astronomical telescopes — has been applied to The latter is seldom achieved for most biological samples, optical microscopy to recover diffraction-limited imaging whose very own mixture of ingredients (e.g. water, pro- performance deep within living tissue. In particular, this teins, nuclear acids, and lipids) gives rise to spatial varia- technology has been used to improve image quality and tions in the refractive index. Such inhomogeneities provide a more accurate characterization of both structure and induce wavefront aberrations, leading to a degradation function of neurons in a variety of living organisms. Among its in the resolution and contrast of microscope images that many highlights, adaptive optical microscopy has made it further deteriorates with imaging depth. possible to image large volumes with diffraction-limited resolution in zebrafish larval brains, to resolve dendritic spines By dynamically measuring the accumulated distortion of m over 600 m deep in the mouse brain, and to more accurately light as it travels through inhomogeneous specimens, characterize the orientation tuning properties of thalamic and correcting for it using active optical components, boutons in the primary visual cortex of awake mice. adaptive optics (AO) can recover diffraction-limited per- formance deep within living systems. In the present Addresses review, we outline the fundamental concepts and meth- 1 Janelia Research Campus, Howard Hughes Medical Institute, Ashburn, ods of adaptive optical microscopy, highlighting VA 20147, USA recent applications of this technology to neurobiology. -
Superresolved Multiphoton Microscopy with Spatial Frequency-Modulated Imaging
Superresolved multiphoton microscopy with spatial frequency-modulated imaging Jeffrey J. Fielda,b,c,d,1,2, Keith A. Wernsinga,1, Scott R. Dominguea, Alyssa M. Allende Motze,f, Keith F. DeLucab,c,d, Dean H. Levif, Jennifer G. DeLucab,c,d, Michael D. Younge, Jeff A. Squiere, and Randy A. Bartelsa,c,d,g aDepartment of Electrical and Computer Engineering, Colorado State University, Fort Collins, CO 80523; bDepartment of Biochemistry and Molecular Biology, Colorado State University, Fort Collins, CO 80523; cMicroscope Imaging Network Foundational Core Facility, Colorado State University, Fort Collins, CO 80523; dInstitute for Genome Architecture and Function, Colorado State University, Fort Collins, CO 80523; eDepartment of Physics, Colorado School of Mines, Golden, CO 80401; fNational Renewable Energy Laboratory, Golden, CO 80401; and gSchool of Biomedical Engineering, Colorado State University, Fort Collins, CO 80523 Edited by Rebecca R. Richards-Kortum, Rice University, Houston, TX, and approved April 19, 2016 (received for review February 23, 2016) Superresolved far-field microscopy has emerged as a powerful electronic states (19), and saturation of scattering due to surface tool for investigating the structure of objects with resolution well plasmon resonance (20, 21). below the diffraction limit of light. Nearly all superresolution Conversely, harmonic generation (HG) results in no net energy imaging techniques reported to date rely on real energy states of transfer to the contrast generating molecules. In this case, the fluorescent molecules to circumvent the diffraction limit, prevent- photokinetics are often described via virtual energy states that ing superresolved imaging with contrast mechanisms that occur drive instantaneous coherent nonlinear scattering. The application via virtual energy states, including harmonic generation (HG).