The Cochlear Labyrinth of Krapina Neandertals by Michaela Beals
Total Page:16
File Type:pdf, Size:1020Kb
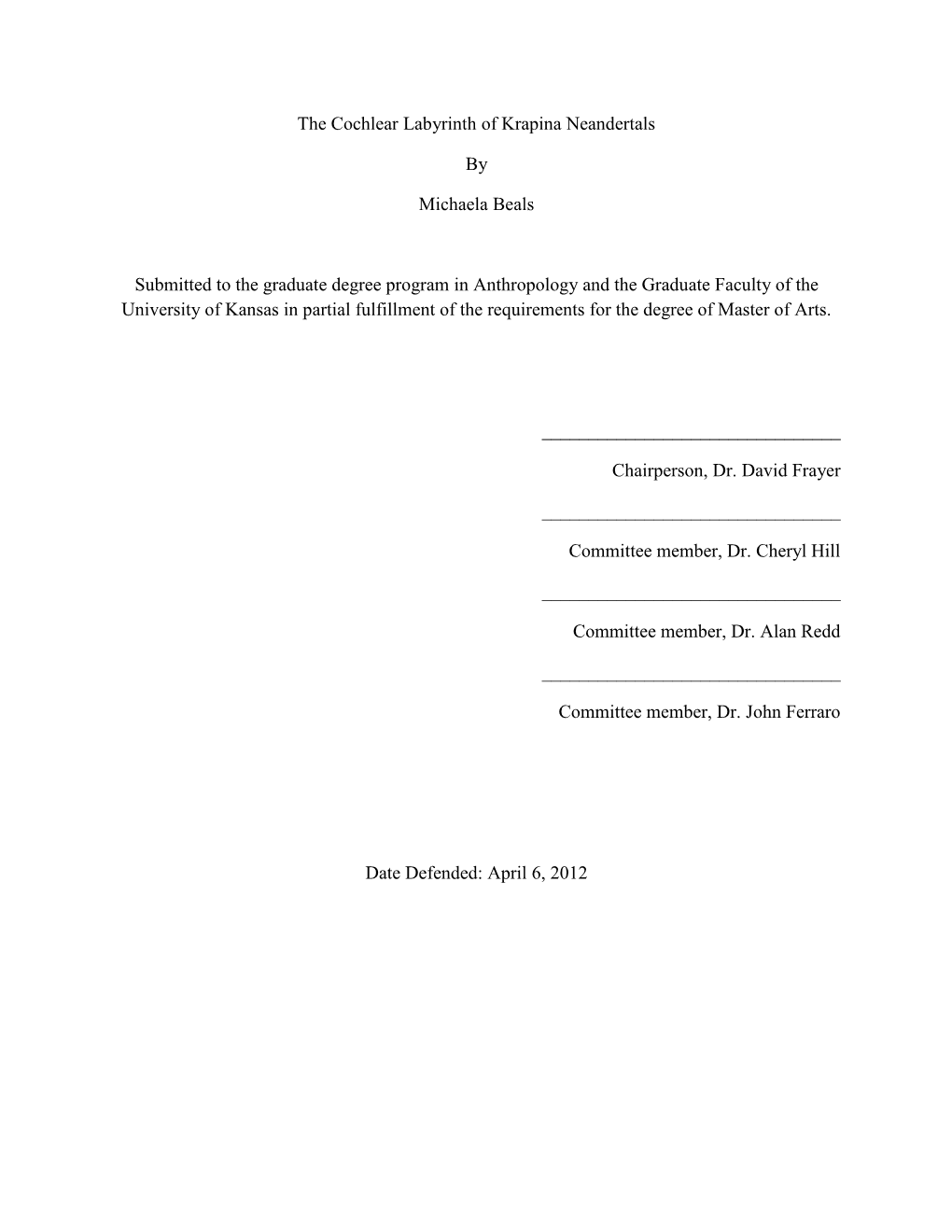
Load more
Recommended publications
-
Fallen in a Dead Ear: Intralabyrinthine Preservation of Stapes in Fossil Artiodactyls Maeva Orliac, Guillaume Billet
Fallen in a dead ear: intralabyrinthine preservation of stapes in fossil artiodactyls Maeva Orliac, Guillaume Billet To cite this version: Maeva Orliac, Guillaume Billet. Fallen in a dead ear: intralabyrinthine preservation of stapes in fossil artiodactyls. Palaeovertebrata, 2016, 40 (1), 10.18563/pv.40.1.e3. hal-01897786 HAL Id: hal-01897786 https://hal.archives-ouvertes.fr/hal-01897786 Submitted on 17 Oct 2018 HAL is a multi-disciplinary open access L’archive ouverte pluridisciplinaire HAL, est archive for the deposit and dissemination of sci- destinée au dépôt et à la diffusion de documents entific research documents, whether they are pub- scientifiques de niveau recherche, publiés ou non, lished or not. The documents may come from émanant des établissements d’enseignement et de teaching and research institutions in France or recherche français ou étrangers, des laboratoires abroad, or from public or private research centers. publics ou privés. See discussions, stats, and author profiles for this publication at: https://www.researchgate.net/publication/297725060 Fallen in a dead ear: intralabyrinthine preservation of stapes in fossil artiodactyls Article · March 2016 DOI: 10.18563/pv.40.1.e3 CITATIONS READS 2 254 2 authors: Maeva Orliac Guillaume Billet Université de Montpellier Muséum National d'Histoire Naturelle 76 PUBLICATIONS 745 CITATIONS 103 PUBLICATIONS 862 CITATIONS SEE PROFILE SEE PROFILE Some of the authors of this publication are also working on these related projects: The ear region of Artiodactyla View project Origin, evolution, and dynamics of Amazonian-Andean ecosystems View project All content following this page was uploaded by Maeva Orliac on 14 March 2016. -
CONGENITAL MALFORMATIONS of the INNER EAR Malformaciones Congénitas Del Oído Interno
topic review CONGENITAL MALFORMATIONS OF THE INNER EAR Malformaciones congénitas del oído interno. Revisión de tema Laura Vanessa Ramírez Pedroza1 Hernán Darío Cano Riaño2 Federico Guillermo Lubinus Badillo2 Summary Key words (MeSH) There are a great variety of congenital malformations that can affect the inner ear, Ear with a diversity of physiopathologies, involved altered structures and age of symptom Ear, inner onset. Therefore, it is important to know and identify these alterations opportunely Hearing loss Vestibule, labyrinth to lower the risks of all the complications, being of great importance, among others, Cochlea the alterations in language development and social interactions. Magnetic resonance imaging Resumen Existe una gran variedad de malformaciones congénitas que pueden afectar al Palabras clave (DeCS) oído interno, con distintas fisiopatologías, diferentes estructuras alteradas y edad Oído de aparición de los síntomas. Por lo anterior, es necesario conocer e identificar Oído interno dichas alteraciones, con el fin de actuar oportunamente y reducir el riesgo de las Pérdida auditiva Vestíbulo del laberinto complicaciones, entre otras —de gran importancia— las alteraciones en el área del Cóclea lenguaje y en el ámbito social. Imagen por resonancia magnética 1. Epidemiology • Hyperbilirubinemia Ear malformations occur in 1 in 10,000 or 20,000 • Respiratory distress from meconium aspiration cases (1). One in every 1,000 children has some degree • Craniofacial alterations (3) of sensorineural hearing impairment, with an average • Mechanical ventilation for more than five days age at diagnosis of 4.9 years. The prevalence of hearing • TORCH Syndrome (4) impairment in newborns with risk factors has been determined to be 9.52% (2). -
ANATOMY of EAR Basic Ear Anatomy
ANATOMY OF EAR Basic Ear Anatomy • Expected outcomes • To understand the hearing mechanism • To be able to identify the structures of the ear Development of Ear 1. Pinna develops from 1st & 2nd Branchial arch (Hillocks of His). Starts at 6 Weeks & is complete by 20 weeks. 2. E.A.M. develops from dorsal end of 1st branchial arch starting at 6-8 weeks and is complete by 28 weeks. 3. Middle Ear development —Malleus & Incus develop between 6-8 weeks from 1st & 2nd branchial arch. Branchial arches & Development of Ear Dev. contd---- • T.M at 28 weeks from all 3 germinal layers . • Foot plate of stapes develops from otic capsule b/w 6- 8 weeks. • Inner ear develops from otic capsule starting at 5 weeks & is complete by 25 weeks. • Development of external/middle/inner ear is independent of each other. Development of ear External Ear • It consists of - Pinna and External auditory meatus. Pinna • It is made up of fibro elastic cartilage covered by skin and connected to the surrounding parts by ligaments and muscles. • Various landmarks on the pinna are helix, antihelix, lobule, tragus, concha, scaphoid fossa and triangular fossa • Pinna has two surfaces i.e. medial or cranial surface and a lateral surface . • Cymba concha lies between crus helix and crus antihelix. It is an important landmark for mastoid antrum. Anatomy of external ear • Landmarks of pinna Anatomy of external ear • Bat-Ear is the most common congenital anomaly of pinna in which antihelix has not developed and excessive conchal cartilage is present. • Corrections of Pinna defects are done at 6 years of age. -
The Temporal Bone
Anatomic Moment The Temporal Bone Joel D. Swartz, David L. Daniels, H. Ric Harnsberger, Katherine A. Shaffer, and Leighton Mark Despite the advent of thin-section high-reso- the inner ear structures and the external lution computed tomography and, more re- environment. cently, unique magnetic resonance imaging se- Virtually all textbooks define the inner ear as quences with thin sections, T2 weighting, and a membranous labyrinth housed within an os- maximum-intensity projection techniques, seous labyrinth. The membranous labyrinth three-dimensional neuroanatomy of the tempo- contains endolymph, a fluid rich in potassium ral bone and related structures remains some- and low in sodium, similar to intracellular fluid. what of an enigma to many medical specialists, Interposed between the membranous labyrinth neuroradiologists included. Therefore, we are and the osseous labyrinth resides a supportive undertaking a series of anatomic moments in perilymphatic labyrinth. Perilymph is similar to the hope of solidifying the most important ana- cerebrospinal fluid and other extracellular tissue tomic concepts as they relate to this region. Our fluid. approach will be organized so as to consider the The osseous labyrinth consists of the bony temporal bone to represent the complex inter- edifice for the vestibule, semicircular canals, relationship of three systems: hearing, balance, cochlea, and vestibular aqueduct. The mem- and related neuroanatomic and neurovascular branous labyrinth consists of the utricle and structures. saccule (located within the vestibule), the semi- The ear originated in fish as a water motion circular ducts, the cochlear duct, and the en- detection system (1). The vestibular (balance) dolymphatic duct. The latter is a channel within mechanism becomes more complex as we the vestibular aqueduct with communications to scale the embryologic ladder and endolymph the utricle and saccule. -
Anatomic Moment
Anatomic Moment Hearing, I: The Cochlea David L. Daniels, Joel D. Swartz, H. Ric Harnsberger, John L. Ulmer, Katherine A. Shaffer, and Leighton Mark The purpose of the ear is to transform me- cochlear recess, which lies on the medial wall of chanical energy (sound) into electric energy. the vestibule (Fig 3). As these sound waves The external ear collects and directs the sound. enter the perilymph of the scala vestibuli, they The middle ear converts the sound to fluid mo- are transmitted through the vestibular mem- tion. The inner ear, specifically the cochlea, brane into the endolymph of the cochlear duct, transforms fluid motion into electric energy. causing displacement of the basilar membrane, The cochlea is a coiled structure consisting of which stimulates the hair cell receptors of the two and three quarter turns (Figs 1 and 2). If it organ of Corti (Figs 4–7) (4, 5). It is the move- were elongated, the cochlea would be approxi- ment of hair cells that generates the electric mately 30 mm in length. The fluid-filled spaces potentials that are converted into action poten- of the cochlea are comprised of three parallel tials in the auditory nerve fibers. The basilar canals: an outer scala vestibuli (ascending spi- membrane varies in width and tension from ral), an inner scala tympani (descending spi- base to apex. As a result, different portions of ral), and the central cochlear duct (scala media) the membrane respond to different auditory fre- (1–7). The scala vestibuli and scala tympani quencies (2, 5). These perilymphatic waves are contain perilymph, a substance similar in com- transmitted via the apex of the cochlea (helico- position to cerebrospinal fluid. -
B005DTJC8Y.Usermanual.Pdf
V2010 Auris Latin Auris externa 1 Auricula 2 Meatus acusticus externus 3 Membrana tympanica Auris media 4 Ossicula auditus: 4 a Malleus 4 b Incus 4 c Stapes 5 Cavitas tympani 6 Tuba auditiva 7 Fenestra vestibuli 8 Fenestra cochleae Auris interna 9 Labyrinthus osseus: 9 a Canalis semicircularis posterior 9 b Canalis semicircularis lateralis 9 c Canalis semicircularis anterior 9 d Vestibulum 9 e Cochlea 10 N. vestibularis 11 N. cochlearis ® 12 N. vestibulocochlearis [VIII] 13 Lig. spirale 14 Scala vestibuli 15 Ductus cochlearis 16 Scala tympani 17 N. cochlearis 18 Ganglion cochleare 19 Organum spirale 20 Modiolus cochleae 21 Paries vestibularis 22 Sulcus spiralis internus 23 Membrana tectoria 24 Cuniculus medius 25 Cuniculus externus 26 Cellulae terminales externae 27 Cellulae sustentaculares externae 28 Sulcus spiralis externus 29 Lamina basilaris 30 Cellulae phalangea externae 31 Cellulae capillares externae 32 Cellula stela externa 33 Cuniculus internus 34 Cellula capillaris interna 35 Cellula stela interna 36 Lamina spiralis ossea 37 Limbus spiralis English The Ear A Sectional view of the human ear B Sectional view of cochlea C Sectional view of cochlear duct with spiral organ External ear 1 Auricle 2 External acoustic meatus 3 Tympanic membrane Middle ear 4 Auditory ossicles: 4 a Malleus 4 b Incus 4 c Stapes 5 Tympanic cavity 6 Pharyngotympanice tube 7 Oval window 8 Round window Internal ear 9 Bony labyrinth 9 a Posterior semicircular canal 9 b Lateral semicircular canal 9 c Anterior semicircular canal ® 9 d Vestibule 9 e Cochlea 10 -
The Nervous System: General and Special Senses
18 The Nervous System: General and Special Senses PowerPoint® Lecture Presentations prepared by Steven Bassett Southeast Community College Lincoln, Nebraska © 2012 Pearson Education, Inc. Introduction • Sensory information arrives at the CNS • Information is “picked up” by sensory receptors • Sensory receptors are the interface between the nervous system and the internal and external environment • General senses • Refers to temperature, pain, touch, pressure, vibration, and proprioception • Special senses • Refers to smell, taste, balance, hearing, and vision © 2012 Pearson Education, Inc. Receptors • Receptors and Receptive Fields • Free nerve endings are the simplest receptors • These respond to a variety of stimuli • Receptors of the retina (for example) are very specific and only respond to light • Receptive fields • Large receptive fields have receptors spread far apart, which makes it difficult to localize a stimulus • Small receptive fields have receptors close together, which makes it easy to localize a stimulus. © 2012 Pearson Education, Inc. Figure 18.1 Receptors and Receptive Fields Receptive Receptive field 1 field 2 Receptive fields © 2012 Pearson Education, Inc. Receptors • Interpretation of Sensory Information • Information is relayed from the receptor to a specific neuron in the CNS • The connection between a receptor and a neuron is called a labeled line • Each labeled line transmits its own specific sensation © 2012 Pearson Education, Inc. Interpretation of Sensory Information • Classification of Receptors • Tonic receptors -
A Place Principle for Vertigo
Auris Nasus Larynx 35 (2008) 1–10 www.elsevier.com/locate/anl A place principle for vertigo Richard R. Gacek * Department of Otolaryngology, Head and Neck Surgery, University of Massachusetts Medical School, Worcester, MA 01655, USA Received 16 March 2007; accepted 13 April 2007 Available online 24 October 2007 Abstract Objective: To provide a road map of the vestibular labyrinth and its innervation leading to a place principle for different forms of vertigo. Method: The literature describing the anatomy and physiology of the vestibular system was reviewed. Results: Different forms of vertigo may be determined by the type of sense organ, type of ganglion cell and location in the vestibular nerve. Conclusion: Partial lesions (viral) of the vestibular ganglion are manifested as various forms of vertigo. # 2007 Elsevier Ireland Ltd. All rights reserved. Keywords: Vertigo; Vestibular nerve; Pathology Contents 1. Introduction . ............................................................................... 1 2. Sense organ. ............................................................................... 2 3. Ganglion cells ............................................................................... 4 4. Hair cells . ............................................................................... 5 5. Hair cell polarization . ....................................................................... 5 6. Efferent vestibular system ....................................................................... 8 7. A place principle for vertigo . ................................................................. -
Inner Ear Defects and Hearing Loss in Mice Lacking the Collagen Receptor
Laboratory Investigation (2008) 88, 27–37 & 2008 USCAP, Inc All rights reserved 0023-6837/08 $30.00 Inner ear defects and hearing loss in mice lacking the collagen receptor DDR1 Angela M Meyer zum Gottesberge1, Oliver Gross2, Ursula Becker-Lendzian1, Thomas Massing1 and Wolfgang F Vogel3 Discoidin domain receptor 1 (DDR1) is a tyrosine kinase receptor that is activated by native collagen. The physiological functions of DDR1 include matrix homeostasis and cell growth, adhesion, branching, and migration, but the specific role of DDR1 in the development and function of the inner ear has not been analyzed. Here, we show that deletion of the DDR1 gene in mouse is associated with a severe decrease in auditory function and substantial structural alterations in the inner ear. Immunohistochemical analysis demonstrated DDR1 expression in several locations in the cochlea, mostly associated with basement membrane and fibrillar collagens; in particular in basal cells of the stria vascularis, type III fibrocytes, and cells lining the basilar membrane of the organ of Corti. In the stria vascularis, loss of DDR1 function resulted in altered morphology of the basal cells and accumulation of electron-dense matrix within the strial epithelial layer in conjunction with a focal and progressive deterioration of strial cells. Cell types in proximity to the basilar membrane, such as Claudius’, inner and outer sulcus cells, also showed marked ultrastructural alterations. Changes in the organ of Corti, such as deterioration of the supporting cells, specifically the outer hair cells, Deiters’, Hensen’s and bordering cells, are likely to interfere with mechanical properties of the organ and may be responsible for the hearing loss observed in DDR1-null mice. -
ACTIVITY 5A STUDENT HANDOUT Glossary: Description and Function of Parts of the Human Ear the Three Functions of the Middle
ACTIVITY 5A STUDENT HANDOUT Glossary: Description and Function of Parts of the Human Ear EXTERNAL EAR AURICLE: the ear flap, ear lobe, or outer ear (pinna); collects sound waves and transmits them through the external acoustic meatus (auditory canal) to the tympanic membrane. EXTERNAL AUDITORY CANAL : an S-shaped structure about 2 cm in length, lined with numerous glands secreting a yellow, waxy substance, cerumen. CERUMEN: yellow, waxy substance; lubricates and protects the ear; “ear wax.” MIDDLE EAR TYMPANIC CAVITY middle: ear, tiny cavity in the temporal bone; holds the three auditory ossicles; has five openings (opening covered by the tympanic membrane), the opening of the auditory tube (eustachian tube) which connects the middle ear with the nasopharynx and through which outside air can enter; the opening into the mastoid cavity, the openings in to the inner ear (round and oval windows). TYMPANIC MEMBRANE: eardrum. AUDITORY OSSICLES: three tiny bones of middle ear, including malleus (hammer), incus (anvil), and stapes (stirrup). STAPEDIUS MUSCLE: attached to the stapes. TENSOR TYMPANI MUSCLE: attached to the handle of the malleus. EUSTACHIAN TUBE: connects middle ear with mouth to equalize pressure. The three functions of the Middle Ear 1. TRANSMIT ENERGY from sound vibrations in the air column of the external auditory meatus across the middle ear into the fluid contained within the cochlea (central hearing apparatus); bones of middle ear pick up the vibrations from the tympanicmembrane and transmit them across the middle ear to the oval window (the opening to the inner ear). 2. PROTECTIVE: reduces the amplitude of vibrations accompanying intense sounds of low frequency; contraction of the tensor tympani and the stapedius restricts the motion of the chain of ossicles and minimizes shock to the inner ear. -
In Vitro Gentamicin Exposure Alters Caveolae Protein Profile in Cochlear Spiral Ligament Pericytes Elisa Ghelfi1* , Yohann Grondin1, Emil J
Ghelfi et al. Proteome Science (2018) 16:7 https://doi.org/10.1186/s12953-018-0132-x RESEARCH Open Access In vitro gentamicin exposure alters caveolae protein profile in cochlear spiral ligament pericytes Elisa Ghelfi1* , Yohann Grondin1, Emil J. Millet1, Adam Bartos1, Magda Bortoni1, Clara Oliveira Gomes dos Santos1,2, Humberto J. Trevino-Villarreal3, Rosalinda Sepulveda1,4 and Rick Rogers1 Abstract Background: The aminoglycoside antibiotic gentamicin is an ototoxic drug and has been used experimentally to investigate cochlear damage induced by noise. We have investigated the changes in the protein profile associated with caveolae in gentamicin treated and untreated spiral ligament (SL) pericytes, specialized cells in the blood labyrinth barrier of the inner ear microvasculature. Pericytes from various microvascular beds express caveolae, protein and cholesterol rich microdomains, which can undergo endocytosis and transcytosis to transport small molecules in and out the cells. A different protein profile in transport-specialized caveolae may induce pathological changes affecting the integrity of the blood labyrinth barrier and ultimately contributing to hearing loss. Method: Caveolae isolation from treated and untreated cells is achieved through ultracentrifugation of the lysates in discontinuous gradients. Mass spectrometry (LC-MS/MS) analysis identifies the proteins in the two groups. Proteins segregating with caveolae isolated from untreated SL pericytes are then compared to caveolae isolated from SL pericytes treated with the gentamicin for 24 h. Data are analyzed using bioinformatic tools. Results: The caveolae proteome in gentamicin treated cells shows that 40% of total proteins are uniquely associated with caveolae during the treatment, and 15% of the proteins normally associated with caveolae in untreated cell are suppressed. -
Anatomy of the Ear
Anatomy of the Ear Lecture (10) ▪ Important ▪ Doctors Notes Please check our Editing File ▪ Notes/Extra explanation ه هذا العمل مب ين بشكل أسا يس عىل عمل دفعة 436 مع المراجعة { َوَم نْ يَ َت َو َ ّكْ عَ َلْ ا َّْلل فَهُ َوْ َحْ سْ ُ ُُْ} والتدقيق وإضافة المﻻحظات وﻻ يغ ين عن المصدر اﻷسا يس للمذاكرة Objectives By the end of the lecture the student should be able to: ✓ List the parts of the ear: External, Middle (tympanic cavity) and Internal (labyrinth). ✓ Describe the parts of the external ear: auricle and external auditory meatus. ✓ Identify the boundaries of the middle ear : roof, floor and four walls (anterior, posterior, medial and lateral). ✓ Define the contents of the tympanic cavity: I. Ear ossicles,: (malleus, incus and stapes) II. Muscles, (tensor tympani and stapedius). III. Nerves (branches of facial and glossopharyngeal). ✓ List the parts of the inner ear, bony part filled with perilymph (Cochlea, vestibule and semicircular canals), in which is suspended the membranous part that filled with endolymph). ✓ List the organs of hearing and equilibrium. External Ear 05:18 o It is formed of the auricle, & the external auditory meatus. o The Auricle has a characteristic shape and collects air vibrations reception of sound. o It consists of a thin plate of elastic cartilage covered by a double layer of skin. o It receives the insertion of extrinsic muscles*, which are supplied by the facial nerve. o Sensation is carried by great auricular (from cervical plexus) & auriculotemporal (from mandibular) nerves. *these muscles are insignificant in humans because they don’t move but are prominent in animals, example: bunnies Extra External Ear o The external auditory canal is a curved S-shaped tube about 2.5cm (one inch), that conducts & collects sound waves from the auricle to the tympanic membrane.