KIM-DISSERTATION.Pdf
Total Page:16
File Type:pdf, Size:1020Kb
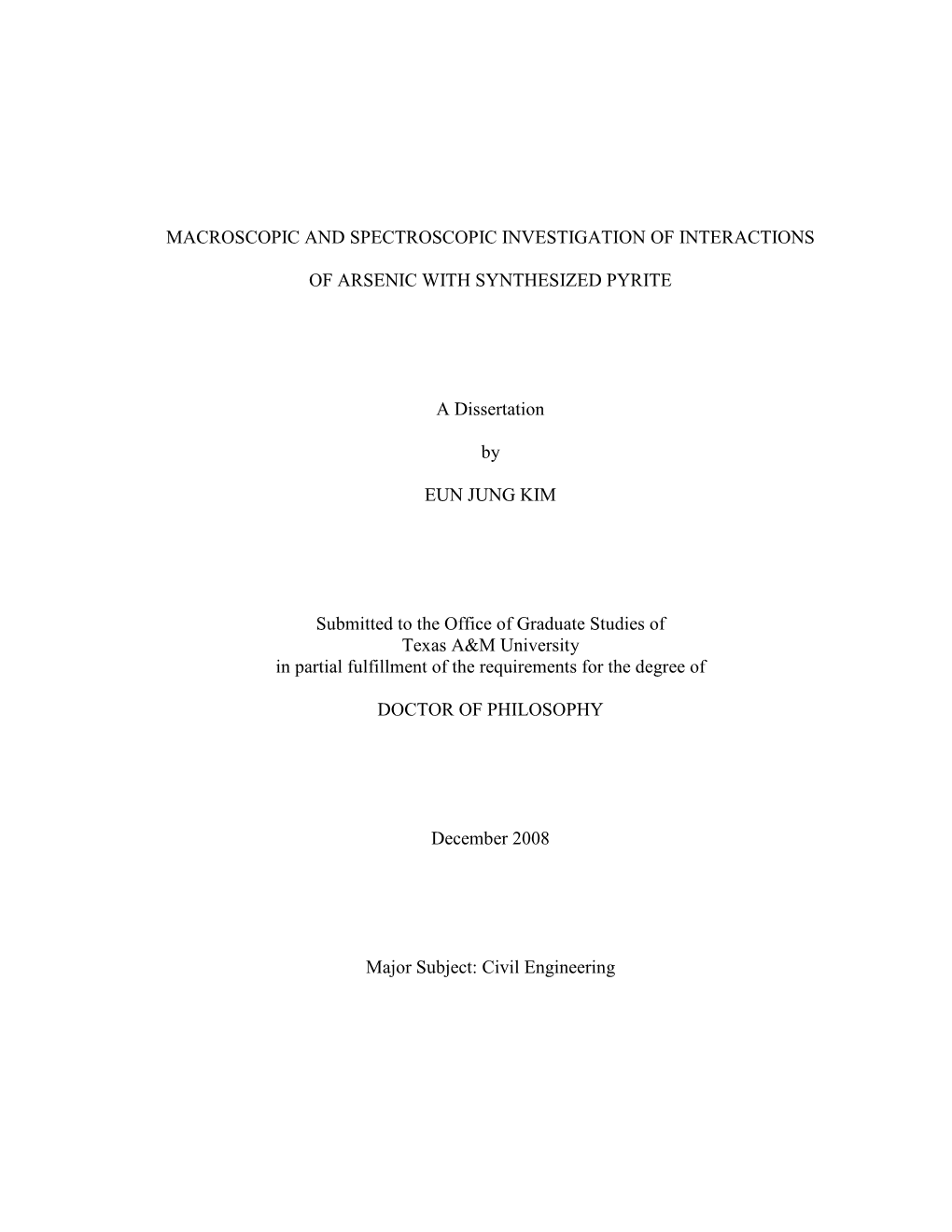
Load more
Recommended publications
-
Washington State Minerals Checklist
Division of Geology and Earth Resources MS 47007; Olympia, WA 98504-7007 Washington State 360-902-1450; 360-902-1785 fax E-mail: [email protected] Website: http://www.dnr.wa.gov/geology Minerals Checklist Note: Mineral names in parentheses are the preferred species names. Compiled by Raymond Lasmanis o Acanthite o Arsenopalladinite o Bustamite o Clinohumite o Enstatite o Harmotome o Actinolite o Arsenopyrite o Bytownite o Clinoptilolite o Epidesmine (Stilbite) o Hastingsite o Adularia o Arsenosulvanite (Plagioclase) o Clinozoisite o Epidote o Hausmannite (Orthoclase) o Arsenpolybasite o Cairngorm (Quartz) o Cobaltite o Epistilbite o Hedenbergite o Aegirine o Astrophyllite o Calamine o Cochromite o Epsomite o Hedleyite o Aenigmatite o Atacamite (Hemimorphite) o Coffinite o Erionite o Hematite o Aeschynite o Atokite o Calaverite o Columbite o Erythrite o Hemimorphite o Agardite-Y o Augite o Calciohilairite (Ferrocolumbite) o Euchroite o Hercynite o Agate (Quartz) o Aurostibite o Calcite, see also o Conichalcite o Euxenite o Hessite o Aguilarite o Austinite Manganocalcite o Connellite o Euxenite-Y o Heulandite o Aktashite o Onyx o Copiapite o o Autunite o Fairchildite Hexahydrite o Alabandite o Caledonite o Copper o o Awaruite o Famatinite Hibschite o Albite o Cancrinite o Copper-zinc o o Axinite group o Fayalite Hillebrandite o Algodonite o Carnelian (Quartz) o Coquandite o o Azurite o Feldspar group Hisingerite o Allanite o Cassiterite o Cordierite o o Barite o Ferberite Hongshiite o Allanite-Ce o Catapleiite o Corrensite o o Bastnäsite -
Theoretical Studies on As and Sb Sulfide Molecules
Mineral Spectroscopy: A Tribute to Roger G. Bums © The Geochemical Society, Special Publication No.5, 1996 Editors: M. D. Dyar, C. McCammon and M. W. Schaefer Theoretical studies on As and Sb sulfide molecules J. A. TOSSELL Department of Chemistry and Biochemistry University of Maryland, College Park, MD 20742, U.S.A. Abstract-Dimorphite (As4S3) and realgar and pararealgar (As4S4) occur as crystalline solids con- taining As4S3 and As4S4 molecules, respectively. Crystalline As2S3 (orpiment) has a layered structure composed of rings of AsS3 triangles, rather than one composed of discrete As4S6 molecules. When orpiment dissolves in concentrated sulfidic solutions the species produced, as characterized by IR and EXAFS, are mononuclear, e.g. ASS3H21, but solubility studies suggest trimeric species in some concentration regimes. Of the antimony sulfides only Sb2S3 (stibnite) has been characterized and its crystal structure does not contain Sb4S6 molecular units. We have used molecular quantum mechanical techniques to calculate the structures, stabilities, vibrational spectra and other properties of As S , 4 3 As4S4, As4S6, As4SIO, Sb4S3, Sb4S4, Sb4S6 and Sb4SlO (as well as S8 and P4S3, for comparison with previous calculations). The calculated structures and vibrational spectra are in good agreement with experiment (after scaling the vibrational frequencies by the standard correction factor of 0.893 for polarized split valence Hartree-Fock self-consistent-field calculations). The calculated geometry of the As4S. isomer recently characterized in pararealgar crystals also agrees well with experiment and is calculated to be about 2.9 kcal/mole less stable than the As4S4 isomer found in realgar. The calculated heats of formation of the arsenic sulfide gas-phase molecules, compared to the elemental cluster molecules As., Sb, and S8, are smaller than the experimental heats of formation for the solid arsenic sulfides, but shown the same trend with oxidation state. -
REVISION 1 an Insight at the Inverse Transformation of Realgar Altered By
REVISION 1 An insight at the inverse transformation of realgar altered by light Giovanni Pratesi1,2 and Matteo Zoppi1* 1Museo di Storia Naturale - Sezione Mineralogia e Litologia, Università di Firenze, via La Pira 4, I- 50121 Firenze, Italy. 2Dipartimento di Scienze della Terra, Università di Firenze, via La Pira 4, I-50121 Firenze, Italy. * E-mail: [email protected] ABSTRACT The light-induced alteration of realgar and β-As4S4 is a well known phenomenon but still displays interesting aspects not completely explained. In the transformation from realgar to pararealgar the molecule As4S4 undergoes a structural modification, and ever since the initial studies two important issues have been highlighted: the influence of the oxygen and the reversibility of the process. The previous study on the reversibility of the altered β-As4S4 points out that this polymorph exhibits a dual behaviour. When the light-induced alteration occurs with the presence of the air, pararealgar and arsenolite, along with amorphous material, are the products, while if the air is not present β-As4S4 turns completely into pararealgar. Moreover, when annealing the altered material in the realgar stability fields (220 °C), in the first case pararealgar and amorphous material turn into stoichiometric alacranite, while in the second case the alteration is completely reversible. Similarly, the present study focuses the attention on the question if realgar, when altered by means of the light and when annealed, might behave as β-As4S4 does. These results display that the phenomenon is more complex. The alteration of realgar with the presence of the air yields pararealgar along with arsenolite, a small quantity of uzonite and amorphous material, and when the air is not present pararealgar is the only product. -
Geological Controls on Pit Lake Chemistry: Implications for the Assessment of Water Quality in Inactive Open Pits
IMWA Proceedings 1998 | © International Mine Water Association 2012 | www.IMWA.info Geological Controls on Pit Lake Chemistry: Implications for the assessment of Water Quality in Inactive Open Pits 1 2 2 2 3 R.J.Bowell , J. Barta , M.Gringrich , W.Mansanares and J. Parshley 'Steffen, Robertson & Kirsten (UK) Limited, Stunmit House, 9 Windsor Place, CardiffCFI 3BX, Wales 2Getchell Gold Corporation, Golconda, Nevada NV89414, USA 3Steffen, Robertson & Kirsten (US) Inc., 1755 E. Plumb Lane, Reno, Nevada NV89502, USA ABSTRACT Open pitting is a method of mining used to exploit many mineral commodities. In many instances mining occurs below the water table leading to active dewatering of the pit during mining. On suspension of activity, pumping is terminated and as infilling of the pit is not always economic the pit is flooded, forming a pit lake. Pit Lakes are viewed as a significant environmental issue owing to their visible presence. It is often, erroneously assumed that all pit lakes will be acid and of poor quality. Consequently in many cases extensive water quality modelling is undertaken to determine the chemical characteristics of a pit lake, with only scant geological knowledge of the pit walls. However, the water quality of a mine pit lake is largely predictable from geological knowledge of the ore deposit, the host rocks and mineralogy. In this paper we present a case study from Nevada of an inactive open pit on which a detailed study was conducted to determine the environmental impacts. This study was greatly enhanced by using geological and geochemical mapping of the pit walls in order to determine controls on pit lake chemistry. -
Growth and Raman Spectroscopic Characterization of As4s4 (II) Single Crystals
*4. Manuscript Click here to view linked References Growth and Raman spectroscopic characterization of As4S4 (II) single crystals Atsushi Kyono Division of Earth Evolution Sciences, Graduate School of Life and Environmental Sciences, University of Tsukuba, 1-1-1 Tennodai Tsukuba, Ibaraki 305-8572, Japan Corresponding author: A. Kyono Present Address: Geophysical Laboratory, Carnegie Institution of Washington, 5251 Broad Branch Rd. NW, Washington, DC 20015-1305, USA Email: [email protected] Phone: +1-202-478-8940, Fax: +1-202-478-8901 pg. 1 ABSTRACT As described by Kutoglu (1976), single crystals of As4S4 (II) phase have been grown using a new two-step synthesis that drastically increases the reproducibility that is attainable in synthetic experiments. First, through photoinduced phase transformation, pararealgar powder is prepared as a precursor instead of AsS melt. Then it is dissolved and recrystallized from CS2 solvent. Results show that single crystals of the As4S4 (II) phase were obtained reproducibly through the dissolution–recrystallization process. Single crystals of As4S4 (II) obtained using the method were translucent and showed a uniform yellow–orange color. The crystal exhibits a platelet-like shape as a thin film with well-developed faces (0 1 0) and (0 -1 0). The grown crystals are as large as 0.50 × 0.50 × 0.01 mm. They were characterized using powder and single crystal X-ray diffraction techniques to confirm the phase identification and the lattice parameters. The As4S4 (II) phase crystallizes in monoclinic system with cell parameters a = 11.202(4) Å, b = 9.954(4) Å, c = 7.142(4) Å, β = 92.81(4)°, V = 795.4(6) Å3, which shows good agreement with the former value. -
Download Download
338 Geologica Macedonica, Vol. 32, No. 2, pp. 95–117 (2018) GEOME 2 IISSN 0352 – 1206 Manuscript received: August 5, 2018 e-ISSN 1857 – 8586 Accepted: November 7, 2018 UDC: 553.46:550.43.08]:504(497.721) 553.497:550.43.08]:504(497.721) Original scientific paper SUPERGENE MINERALOGY OF THE LOJANE Sb-As-Cr DEPOSIT, REPUBLIC OF MACEDONIA: TRACING THE MOBILIZATION OF TOXIC METALS Uwe Kolitsch1,2, Tamara Đorđević2, Goran Tasev3, Todor Serafimovski3, Ivan Boev3, Blažo Boev3 1Mineralogisch-Petrographische Abt., Naturhistorisches Museum, Burgring 7, A-1010 Wien, Austria 2Institut für Mineralogie und Kristallographie, Universität Wien, Althanstr. 14, A-1090 Wien, Austria 3Department of Mineral Deposits, Faculty of Natural and Technical Sciences, “Goce Delčev” University in Štip, Blvd. Goce Delčev 89, 2000 Štip, Republic of Macedonia [email protected] A b s t r a c t: As part of a larger project on the environmental mineralogy and geochemistry of the Lojane Sb- As-Cr deposit, Republic of Macedonia, which was mined for chromite and, later, stibnite until 1979 and is a substantial source of arsenic and antimony pollution, the supergene mineralogy of the deposit was studied. Samples collected on ore and waste dumps were used to identify and characterize the previously uninvestigated suite of supergene mineral phases by standard mineralogical techniques. The following species were determined (in alphabetical order): annaber- gite, arseniosiderite(?), gypsum, hexahydrite, hörnesite, pararealgar, roméite-group minerals, rozenite, scorodite, sen- armontite, stibiconite, sulphur, tripuhyite and valentinite. Their occurrences are described and their local conditions of formation are discussed. High-resolution Raman spectra of hörnesite, hexahydrite and rozenite are provided and com- pared with literature data. -
Allchar Mineral Assemblage 3
Geologica Macedonica, Vol. 15-16, p. 1-23 (2001-2002) Suppl. GEOME2 SSN 0352 - 1206 Manuscript received: December 15, 200 1 UDC: 553.08 (497 .7) Accepted: March 3, 2002 Original scientific paper ~• ALLCHAR l\1INERAL ASSEMBLAGE l 3 l 4 Blazo Boev , Vladimir Bermanec , Todor Serafimovski\ Sonja Lepitkova , Snezana Mikulcic , 5 5 5 Marin ~oufek\ Gligor Jovanovski , Trajce Stafilov , Metodija Najdoski IDepartment ofPetrology, Mineralogy and Geochemistry, Faculty ofMining and Geology, "Sv. Kiril i Metodij" University, Goce Delcev 89, MK-2000 Stip, Republic ofMacedonia 2Department ofMineral Deposits, Faculty ofMining and Geology, "Sv. Kiril i Metodij" University, Goce Delcev 89, MK-2000 Stip, Republic of Macedonia 3Institute ofMineralogy and Petrology, Department ofGeology, Faculty ofScience, University ofZagreb, Horvatovac bb, HR-JOOOO Zagreb, Croatia 4Department ofMineralogy and Petrology, Croatian Natural History Museum, Demetrova I, HR-JOOOO Zagreb, Croatia 5Institute of Chemistry, Faculty ofScience, "Sv. Kiril i Metodij" University, P.o. Box 162, MK-JOOI Skopje, Republic ofMacedonia Abstract. The paper is a summary of investigatioris carried out on minerals of the Allchar deposit. It discusses the TI-As-Sb-Au mineral ill emblage after detailed and intense research work. Four types of mineralization have been distinguished based on the mineral assemblage present: I. The first ly pe is characterised by high c ment of iron and sulphur, but lower arsenic and thalli um contenl. The pyrite-marcasite mineral assemblage is characterized by the presence of some quantities of arsenic-pyrite. 2. The second type is characterized by the high antimony and low iron and thallium content. Stibnite is the most common mineral in the group. -
Characterization and Comparative Physico-Chemical Studies Of
RESEARCH COMMUNICATIONS 19. Peng, L. and Yu, B., Numerical study of regional environmental Characterization and comparative carrying capacity for livestock and poultry farming based on planting-breeding balance. J. Environ. Sci., 2013, 25(9), 1882– physico-chemical studies of 1889. 20. State Environmental Protection Administration, Investigation and Manahshila (traditionally used arsenic Control Counter Measures of Pollution Situation of Livestock and mineral) and the corresponding Poultry Breeding Industry in China, China Environmental Science Press, Beijing, 2002, pp. 14–103. polymorphs of realgar (As4S4) 21. Peng, L. and Wang, D., Estimation of annual quantity of total excretion from livestock and poultry in Chongqing municipality. 1, 2 Trans. Chin. Soc. Agric. Eng., 2004, 20(1), 288–292. Vinamra Sharma *, Amiya K. Samal , 22. Yan, B. J. and Pan, Y. C., Estimation of nitrogen pollution load of Anand K. Chaudhary1 and Rajesh K. Srivastava2 farmland from livestock manure in China based on grid. Bull. Soil 1Department of Rasa Shastra, Faculty of Ayurveda, Water Conserv., 2015, 35(5), 133–137. Institute of Medical Sciences, and 23. Yan, B. J., Zhao, C. J., Pan, Y. C. and Wang, Y., Estimation of the 2Centre of Advanced Study in Geology, Institute of Science, amount of livestock manure and its environmental influence of Banaras Hindu University, Varanasi 221 005, India large-scaled culture based on spatial information. China Environ. Sci., 2009, 29(7), 733–737. 24. Yan, B. J., Zhao, C. J., Pan, Y. C., Yan, J. J. and Guo, X., Estima- This communication presents characterization and tion of livestock manure nitrogen load and pollution risk evalua- comparison of the physico-chemical properties of dif- tion of farmland in Daxing District. -
Thermal Behavior of Realgar As4s4, and of Arsenolite As2o3 and Non-Stoichiometric As8s8+X
American Mineralogist, Volume 97, pages 1320–1329, 2012 Thermal behavior of realgar As4S4, and of arsenolite As2O3 and non-stoichiometric As8S8+x crystals produced from As4S4 melt recrystallization PAOLO BALLIRANO* Dipartimento di Scienze della Terra, Sapienza Università di Roma, P.le Aldo Moro 5, I-00185 Roma, Italy ABSTRACT An in situ high-temperature X-ray powder diffraction study of the thermal behavior of realgar (α-As4S4) has been carried out. Data, measured in transmission geometry on a non-hermetically sealed capillary, indicate that the realgar → β-As4S4 phase transition starts at 558 K and is completed at 573 K due to kinetics. Melting starts at 578 K and is completed at 588 K. Thermal expansion of realgar is significant and fairly isotropic. In fact, the a- and b-parameters expand almost at the same rate, whereas the c-parameter is slightly softer against heating. Moreover, the β-angle contracts as temperature is raised. The geometry of the As4S4 molecule is largely independent from heating. The lengthening of a few As-S and As-As contacts above or near the sum of the As,S van der Waals radii represents the driving force of the phase transition. In addition, the thermal behavior of arsenolite As2O3 and non- stoichiometric As8S8+x crystals produced from As4S4 melt recrystallization has been investigated. Two members located along the β-As4S4-alacranite (As8S9) series joint were identified at RT: a term close to the β-As4S4 end-member (As8S8+x: x = ca. 0.1) and one term of approximate As8S8.3 composition. The thermal expansion of β-As4S4 is significantly anisotropic following the αb > αa > αc relationship. -
Light-Induced Molecular Change in Hgi2⋅As4s4
American Mineralogist, Volume 96, pages 646–653, 2011 Light-induced molecular change in HgI2·As4S4: Evidence by single-crystal X-ray diffraction and Raman spectroscopy P. BONAZZI ,1,* L. BINDI ,2 M. MUNIZ -MIRANDA ,3 L. CHE L AZZI ,1 T. RÖD L ,4 AND A. PFI T ZNER 4 1Dipartimento di Scienze della Terra, Università di Firenze, via La Pira 4, I-50121 Firenze, Italy 2Museo di Storia Naturale, Sezione di Mineralogia, Università di Firenze, via La Pira 4, I-50121 Firenze, Italy 3Dipartimento di Chimica, Università di Firenze, via della Lastruccia, 13 I-50019 Sesto F.no, Firenze, Italy 4Institut für Anorganische Chemie, Universität Regensburg, Universitätsstrasse 31, D-93040 Regensburg, Germany AB S T RA CT To investigate the behavior of the As4S4 molecule within a crystal-chemical environment differing from realgar, α-As4S4, and its high-temperature polymorph, β-As4S4, the effects of the light exposure on the structure of the HgI2·As4S4 adduct have been studied. The structure of this compound consists of 3 a packing of nearly linear HgI2 molecules and As4S4 cage-molecules. A crystal [V = 1295.9(4) Å ] was exposed to filtered polychromatic light (550 nm long-wavelength pass filter). A marked increase of the unit-cell volume as a function of the exposure time was observed up to V = 1338.9 Å3 at 3060 min of exposure. Structure refinements indicated that the increase of the unit-cell volume is to ascribe to the formation of an increasing fraction (up to 59%) of pararealgar replacing the realgar-type molecule. After this point, further light-exposure did not cause any further increase of the lattice parameters. -
Anorpiment, As2s3, the Triclinic Dimorph of Orpiment
Mineralogical Magazine, December 2011, Vol. 75(6), pp. 2857–2867 Anorpiment, As2S3, the triclinic dimorph of orpiment 1, 2 3 4 5 A. R. KAMPF *, R. T. DOWNS ,R.M.HOUSLEY ,R.A.JENKINS AND J. HYRSˇL 1 Mineral Sciences Department, Natural History Museum of Los Angeles County, 900 Exposition Boulevard, Los Angeles, California 90007, USA 2 Department of Geosciences, University of Arizona, Tucson, Arizona 85721-0077, USA 3 Division of Geological and Planetary Sciences, California Institute of Technology, Pasadena, CA 91125, USA 4 4521 N. Via Madre, Tucson, AZ 85749, USA 5 Ke kurtum 383, 142 00 Praha 4, Czech Republic [Received 12 September 2011; Accepted 27 October 2011] ABSTRACT The new mineral anorpiment, As2S3, the triclinic dimorph of orpiment, has space group P1¯ and cell parameters a = 5.7577(2), b = 8.7169(3), c = 10.2682(7) A˚ , a = 78.152(7), b = 75.817(7), g = 89.861(6)º, V = 488.38(4) A˚ 3 and Z = 4. It occurs at the Palomo mine, Castrovirreyna Province, Huancavelica Department, Peru. It is a low-temperature hydrothermal mineral associated with dufre´noysite, muscovite, orpiment, pyrite and realgar. It occurs in drusy crusts of wedge-shaped, transparent, greenish yellow crystals. The streak is yellow. The lustre is resinous on crystal faces, but pearly on cleavage surfaces. The Mohs hardness is about 1Ý. The mineral is sectile with an irregular fracture and one perfect and easy cleavage on {001}. The measured and calculated densities are 3.33 and 3.321 g cmÀ3, respectively. All indices of refraction are greater than 2. -
Alacránite As8s9
Alacránite As8S9 Crystal Data: Monoclinic. Point Group: 2/m. Crystals are pinacoidal, prismatic, and flattened on [100], striated ∥ [001] on {100}, to 1 mm; as subhedral flattened prismatic grains. Physical Properties: Cleavage: Imperfect on {100}. Fracture: Conchoidal. Tenacity: Very brittle. Hardness = 1.5 VHN = 69 (20 g load). D(meas.) = 3.43(3) D(calc.) = 3.503 Optical Properties: Transparent to translucent. Color: Orange to pale gray with rose-yellow internal reflections in reflected light; orange-yellow in transmitted light. Streak: Yellow-orange. Luster: Adamantine, vitreous, resinous, greasy. Optical Class: Biaxial (+). α = 2.39(1) β = n.d. γ = 2.52(2) 2V = n.d. R1-R2: (400) 13.0-14.0, (425) 13.2-14.6, (450) 13.3-14.8, (475) 13.4-14.8, (500) 13.3-14.5, (525) 13.1-14.3, (550) 13.2-14.5, (575) 13.4-14.7, (600) 13.5-14.8, (625) 13.6-14.9, (650) 13.7-15.0, (675) 13.8-15.0, (700) 13.9-15.1 Cell Data: Space Group: P2/c. a = 9.943(1) b = 9.366(1) c = 8.908(1) β = 102.007° Z = 2 X-ray Powder Pattern: Uzon caldera, Russia. 3.064 (100), 5.91 (90), 2.950 (90), 5.11 (80), 4.05 (70), 3.291 (50), 6.89 (40) Chemistry: (1) (2) (3) (4) As 67.35 67.80 70.03 67.52 S 32.61 32.20 29.97 32.48 Total 99.96 100.00 100.00 100.00 (1) Uzon caldera, Russia; average of 4 electron microprobe analyses; corresponding to As0.88S1.00.