Journal.Pgen.1006024
Total Page:16
File Type:pdf, Size:1020Kb
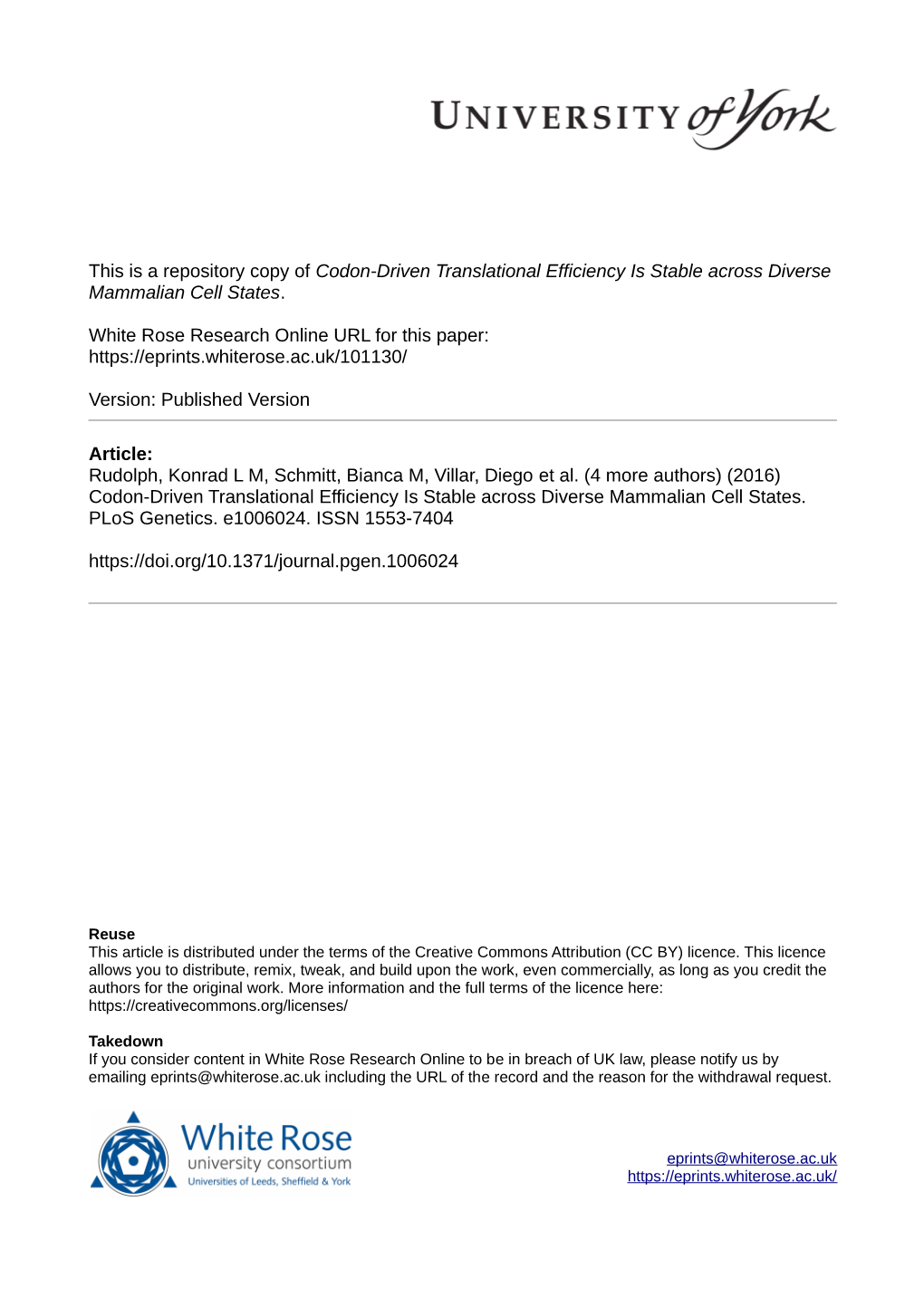
Load more
Recommended publications
-
Proteomics Provides Insights Into the Inhibition of Chinese Hamster V79
www.nature.com/scientificreports OPEN Proteomics provides insights into the inhibition of Chinese hamster V79 cell proliferation in the deep underground environment Jifeng Liu1,2, Tengfei Ma1,2, Mingzhong Gao3, Yilin Liu4, Jun Liu1, Shichao Wang2, Yike Xie2, Ling Wang2, Juan Cheng2, Shixi Liu1*, Jian Zou1,2*, Jiang Wu2, Weimin Li2 & Heping Xie2,3,5 As resources in the shallow depths of the earth exhausted, people will spend extended periods of time in the deep underground space. However, little is known about the deep underground environment afecting the health of organisms. Hence, we established both deep underground laboratory (DUGL) and above ground laboratory (AGL) to investigate the efect of environmental factors on organisms. Six environmental parameters were monitored in the DUGL and AGL. Growth curves were recorded and tandem mass tag (TMT) proteomics analysis were performed to explore the proliferative ability and diferentially abundant proteins (DAPs) in V79 cells (a cell line widely used in biological study in DUGLs) cultured in the DUGL and AGL. Parallel Reaction Monitoring was conducted to verify the TMT results. γ ray dose rate showed the most detectable diference between the two laboratories, whereby γ ray dose rate was signifcantly lower in the DUGL compared to the AGL. V79 cell proliferation was slower in the DUGL. Quantitative proteomics detected 980 DAPs (absolute fold change ≥ 1.2, p < 0.05) between V79 cells cultured in the DUGL and AGL. Of these, 576 proteins were up-regulated and 404 proteins were down-regulated in V79 cells cultured in the DUGL. KEGG pathway analysis revealed that seven pathways (e.g. -
Supplemental Table 1. Complete Gene Lists and GO Terms from Figure 3C
Supplemental Table 1. Complete gene lists and GO terms from Figure 3C. Path 1 Genes: RP11-34P13.15, RP4-758J18.10, VWA1, CHD5, AZIN2, FOXO6, RP11-403I13.8, ARHGAP30, RGS4, LRRN2, RASSF5, SERTAD4, GJC2, RHOU, REEP1, FOXI3, SH3RF3, COL4A4, ZDHHC23, FGFR3, PPP2R2C, CTD-2031P19.4, RNF182, GRM4, PRR15, DGKI, CHMP4C, CALB1, SPAG1, KLF4, ENG, RET, GDF10, ADAMTS14, SPOCK2, MBL1P, ADAM8, LRP4-AS1, CARNS1, DGAT2, CRYAB, AP000783.1, OPCML, PLEKHG6, GDF3, EMP1, RASSF9, FAM101A, STON2, GREM1, ACTC1, CORO2B, FURIN, WFIKKN1, BAIAP3, TMC5, HS3ST4, ZFHX3, NLRP1, RASD1, CACNG4, EMILIN2, L3MBTL4, KLHL14, HMSD, RP11-849I19.1, SALL3, GADD45B, KANK3, CTC- 526N19.1, ZNF888, MMP9, BMP7, PIK3IP1, MCHR1, SYTL5, CAMK2N1, PINK1, ID3, PTPRU, MANEAL, MCOLN3, LRRC8C, NTNG1, KCNC4, RP11, 430C7.5, C1orf95, ID2-AS1, ID2, GDF7, KCNG3, RGPD8, PSD4, CCDC74B, BMPR2, KAT2B, LINC00693, ZNF654, FILIP1L, SH3TC1, CPEB2, NPFFR2, TRPC3, RP11-752L20.3, FAM198B, TLL1, CDH9, PDZD2, CHSY3, GALNT10, FOXQ1, ATXN1, ID4, COL11A2, CNR1, GTF2IP4, FZD1, PAX5, RP11-35N6.1, UNC5B, NKX1-2, FAM196A, EBF3, PRRG4, LRP4, SYT7, PLBD1, GRASP, ALX1, HIP1R, LPAR6, SLITRK6, C16orf89, RP11-491F9.1, MMP2, B3GNT9, NXPH3, TNRC6C-AS1, LDLRAD4, NOL4, SMAD7, HCN2, PDE4A, KANK2, SAMD1, EXOC3L2, IL11, EMILIN3, KCNB1, DOK5, EEF1A2, A4GALT, ADGRG2, ELF4, ABCD1 Term Count % PValue Genes regulation of pathway-restricted GDF3, SMAD7, GDF7, BMPR2, GDF10, GREM1, BMP7, LDLRAD4, SMAD protein phosphorylation 9 6.34 1.31E-08 ENG pathway-restricted SMAD protein GDF3, SMAD7, GDF7, BMPR2, GDF10, GREM1, BMP7, LDLRAD4, phosphorylation -
Egfr Activates a Taz-Driven Oncogenic Program in Glioblastoma
EGFR ACTIVATES A TAZ-DRIVEN ONCOGENIC PROGRAM IN GLIOBLASTOMA by Minling Gao A thesis submitted to Johns Hopkins University in conformity with the requirements for the degree of Doctor of Philosophy Baltimore, Maryland March 2020 ©2020 Minling Gao All rights reserved Abstract Hyperactivated EGFR signaling is associated with about 45% of Glioblastoma (GBM), the most aggressive and lethal primary brain tumor in humans. However, the oncogenic transcriptional events driven by EGFR are still incompletely understood. We studied the role of the transcription factor TAZ to better understand master transcriptional regulators in mediating the EGFR signaling pathway in GBM. The transcriptional coactivator with PDZ- binding motif (TAZ) and its paralog gene, the Yes-associated protein (YAP) are two transcriptional co-activators that play important roles in multiple cancer types and are regulated in a context-dependent manner by various upstream signaling pathways, e.g. the Hippo, WNT and GPCR signaling. In GBM cells, TAZ functions as an oncogene that drives mesenchymal transition and radioresistance. This thesis intends to broaden our understanding of EGFR signaling and TAZ regulation in GBM. In patient-derived GBM cell models, EGF induced TAZ and its known gene targets through EGFR and downstream tyrosine kinases (ERK1/2 and STAT3). In GBM cells with EGFRvIII, an EGF-independent and constitutively active mutation, TAZ showed EGF- independent hyperactivation when compared to EGFRvIII-negative cells. These results revealed a novel EGFR-TAZ signaling axis in GBM cells. The second contribution of this thesis is that we performed next-generation sequencing to establish the first genome-wide map of EGF-induced TAZ target genes. -
Heterogeneity Between Primary Colon Carcinoma and Paired Lymphatic and Hepatic Metastases
MOLECULAR MEDICINE REPORTS 6: 1057-1068, 2012 Heterogeneity between primary colon carcinoma and paired lymphatic and hepatic metastases HUANRONG LAN1, KETAO JIN2,3, BOJIAN XIE4, NA HAN5, BINBIN CUI2, FEILIN CAO2 and LISONG TENG3 Departments of 1Gynecology and Obstetrics, and 2Surgical Oncology, Taizhou Hospital, Wenzhou Medical College, Linhai, Zhejiang; 3Department of Surgical Oncology, First Affiliated Hospital, College of Medicine, Zhejiang University, Hangzhou, Zhejiang; 4Department of Surgical Oncology, Sir Run Run Shaw Hospital, College of Medicine, Zhejiang University, Hangzhou, Zhejiang; 5Cancer Chemotherapy Center, Zhejiang Cancer Hospital, Zhejiang University of Chinese Medicine, Hangzhou, Zhejiang, P.R. China Received January 26, 2012; Accepted May 8, 2012 DOI: 10.3892/mmr.2012.1051 Abstract. Heterogeneity is one of the recognized characteris- Introduction tics of human tumors, and occurs on multiple levels in a wide range of tumors. A number of studies have focused on the Intratumor heterogeneity is one of the recognized charac- heterogeneity found in primary tumors and related metastases teristics of human tumors, which occurs on multiple levels, with the consideration that the evaluation of metastatic rather including genetic, protein and macroscopic, in a wide range than primary sites could be of clinical relevance. Numerous of tumors, including breast, colorectal cancer (CRC), non- studies have demonstrated particularly high rates of hetero- small cell lung cancer (NSCLC), prostate, ovarian, pancreatic, geneity between primary colorectal tumors and their paired gastric, brain and renal clear cell carcinoma (1). Over the past lymphatic and hepatic metastases. It has also been proposed decade, a number of studies have focused on the heterogeneity that the heterogeneity between primary colon carcinomas and found in primary tumors and related metastases with the their paired lymphatic and hepatic metastases may result in consideration that the evaluation of metastatic rather than different responses to anticancer therapies. -
Gene Duplication and Neofunctionalization: POLR3G and POLR3GL
Downloaded from genome.cshlp.org on September 27, 2021 - Published by Cold Spring Harbor Laboratory Press Research Gene duplication and neofunctionalization: POLR3G and POLR3GL Marianne Renaud,1 Viviane Praz,1,2 Erwann Vieu,1,5 Laurence Florens,3 Michael P. Washburn,3,4 Philippe l’Hoˆte,1 and Nouria Hernandez1,6 1Center for Integrative Genomics, Faculty of Biology and Medicine, University of Lausanne, 1015 Lausanne, Switzerland; 2Swiss Institute of Bioinformatics, 1015 Lausanne, Switzerland; 3Stowers Institute for Medical Research, Kansas City, Missouri 64110, USA; 4Department of Pathology and Laboratory Medicine, The University of Kansas Medical Center, Kansas City, Kansas 66160, USA RNA polymerase III (Pol III) occurs in two versions, one containing the POLR3G subunit and the other the closely related POLR3GL subunit. It is not clear whether these two Pol III forms have the same function, in particular whether they recognize the same target genes. We show that the POLR3G and POLR3GL genes arose from a DNA-based gene duplication, probably in a common ancestor of vertebrates. POLR3G- as well as POLR3GL-containing Pol III are present in cultured cell lines and in normal mouse liver, although the relative amounts of the two forms vary, with the POLR3G-containing Pol III relatively more abundant in dividing cells. Genome-wide chromatin immunoprecipitations followed by high-throughput sequencing (ChIP-seq) reveal that both forms of Pol III occupy the same target genes, in very constant proportions within one cell line, suggesting that the two forms of Pol III have a similar function with regard to specificity for target genes. In contrast, the POLR3G promoter—not the POLR3GL promoter—binds the transcription factor MYC, as do all other pro- moters of genes encoding Pol III subunits. -
Brain-Region-Specific Contributions of Foxp1 to Autism And
BRAIN-REGION-SPECIFIC CONTRIBUTIONS OF FOXP1 TO AUTISM AND INTELLECTUAL DISABILITY PHENOTYPES Daniel John Araujo APPROVED BY SUPERVISORY COMMITTEE: Amelia J. Eisch, Ph.D. (Chair) _____________________________ Genevieve Konopka, Ph.D. (Mentor) _____________________________ Craig M. Powell, M.D., Ph.D. _____________________________ Lenora J. Volk, Ph.D. _____________________________ Jiang Wu, Ph.D. _____________________________ DEDICATION I would like to thank my thesis advisor Dr. Genevieve Konopka who has always pushed me to strive for excellence in my work. It’s been an honor to study neuroscience under her supervision and she will serve as an example for the rest of my scientific career. I would like to thank my wife Sarah Teresita Vega for her unwavering love and patience. Sarah has been my best friend for the past nine years and her encouragement has allowed me to face any challenge with grit. I also thank my thesis committee members for all of their suggestions on how to improve my research. I would like to thank Dr. Timothy Raabe and Dr. Nancy Street for their continuous support of both my undergraduate and graduate career. Finally, I dedicate this dissertation to my grandparents George Cuellar Araujo and Margarita Salinas Araujo who raised my two siblings and me. Any success I’ve had in life belongs to them. ii BRAIN-REGION-SPECIFIC CONTRIBUTIONS OF FOXP1 TO AUTISM AND INTELLECTUAL DISABILITY PHENOTYPES By DANIEL JOHN ARAUJO, B.S. DISSERTATION Presented to the Faculty of the Graduate School of Biomedical Sciences at The University of Texas Southwestern Medical Center In Partial Fulfillment of the Requirements for the Degree of DOCTOR OF PHILOSOPHY The University of Texas Southwestern Medical Center Dallas, Texas August 11, 2017 iii Copyright By Daniel John Araujo, 2017 All Rights Reserved iv BRAIN-REGION-SPECIFIC CONTRIBUTIONS OF FOXP1 TO AUTISM AND INTELLECTUAL DISABILITY PHENOTPES Publication No. -
Supplementary Table 1 Double Treatment Vs Single Treatment
Supplementary table 1 Double treatment vs single treatment Probe ID Symbol Gene name P value Fold change TC0500007292.hg.1 NIM1K NIM1 serine/threonine protein kinase 1.05E-04 5.02 HTA2-neg-47424007_st NA NA 3.44E-03 4.11 HTA2-pos-3475282_st NA NA 3.30E-03 3.24 TC0X00007013.hg.1 MPC1L mitochondrial pyruvate carrier 1-like 5.22E-03 3.21 TC0200010447.hg.1 CASP8 caspase 8, apoptosis-related cysteine peptidase 3.54E-03 2.46 TC0400008390.hg.1 LRIT3 leucine-rich repeat, immunoglobulin-like and transmembrane domains 3 1.86E-03 2.41 TC1700011905.hg.1 DNAH17 dynein, axonemal, heavy chain 17 1.81E-04 2.40 TC0600012064.hg.1 GCM1 glial cells missing homolog 1 (Drosophila) 2.81E-03 2.39 TC0100015789.hg.1 POGZ Transcript Identified by AceView, Entrez Gene ID(s) 23126 3.64E-04 2.38 TC1300010039.hg.1 NEK5 NIMA-related kinase 5 3.39E-03 2.36 TC0900008222.hg.1 STX17 syntaxin 17 1.08E-03 2.29 TC1700012355.hg.1 KRBA2 KRAB-A domain containing 2 5.98E-03 2.28 HTA2-neg-47424044_st NA NA 5.94E-03 2.24 HTA2-neg-47424360_st NA NA 2.12E-03 2.22 TC0800010802.hg.1 C8orf89 chromosome 8 open reading frame 89 6.51E-04 2.20 TC1500010745.hg.1 POLR2M polymerase (RNA) II (DNA directed) polypeptide M 5.19E-03 2.20 TC1500007409.hg.1 GCNT3 glucosaminyl (N-acetyl) transferase 3, mucin type 6.48E-03 2.17 TC2200007132.hg.1 RFPL3 ret finger protein-like 3 5.91E-05 2.17 HTA2-neg-47424024_st NA NA 2.45E-03 2.16 TC0200010474.hg.1 KIAA2012 KIAA2012 5.20E-03 2.16 TC1100007216.hg.1 PRRG4 proline rich Gla (G-carboxyglutamic acid) 4 (transmembrane) 7.43E-03 2.15 TC0400012977.hg.1 SH3D19 -
Topics Symposia
Abstracts Topics Symposia Symposia S_1 Structural variation S 1 Structural variation S 2 Low risk cancer genes S1_01 S 3 Transgeneration effects and Molecular Mechanisms and Clinical Consequences of Genomic Disor- epigenetic programming ders. Implementation of array CGH in Genetic Diagnostics. The Baylor S 4 Ciliopathies Experience S 5 Systems biology Pawel Stankiewicz S 6 Molecular processes in meiosis Dept. of Molecular & Human Genetics, Baylor College of Medicine, Hous- ton, TX, USA Genomic disorders are a group of human genetic diseases caused by Selected Presentations DNA rearrangements, ranging in size from an average exon (~100 bp) to megabases and affecting dosage sensitive genes. Three major mo- lecular mechanisms: non-allelic homologous recombination (NAHR), Workshops non-homologous end joining (NHEJ), and the fork stalling and tem- W 1 Clinical genetics plate switching (FoSTeS)/microhomology-mediated breakage-induced W 2 Molecular basis of disease I repair (MMBIR) have been described as causative for the vast majority W 3 Cancer genetics of genomic disorders. Recurrent rearrangements are typically mediated W 4 Molecular basis of disease II by NAHR between low-copy repeats that are usually >10 kb in size with >97% DNA sequence identity. Nonrecurrent CNVs have been found to W 5 Imprinting be formed by NAHR between highly homologous repetitive elements W 6 Genomics technology / bioinformatics (e.g. Alu, LINE) and more often by NHEJ and FoSTeS/MMBIR stimu- W 7 Complex diseases lated, but not mediated, by genomic architectural features. Further- W 8 Cytogenetics / prenatal genetics more, simple repeating DNA sequences that have a potential of adopt- ing non-B DNA conformations (e.g. -
Mitotic Checkpoints and Chromosome Instability Are Strong Predictors of Clinical Outcome in Gastrointestinal Stromal Tumors
MITOTIC CHECKPOINTS AND CHROMOSOME INSTABILITY ARE STRONG PREDICTORS OF CLINICAL OUTCOME IN GASTROINTESTINAL STROMAL TUMORS. Pauline Lagarde1,2, Gaëlle Pérot1, Audrey Kauffmann3, Céline Brulard1, Valérie Dapremont2, Isabelle Hostein2, Agnès Neuville1,2, Agnieszka Wozniak4, Raf Sciot5, Patrick Schöffski4, Alain Aurias1,6, Jean-Michel Coindre1,2,7 Maria Debiec-Rychter8, Frédéric Chibon1,2. Supplemental data NM cases deletion frequency. frequency. deletion NM cases Mand between difference the highest setswith of theprobe a view isdetailed panel Bottom frequently. sorted totheless deleted theprobe are frequently from more and thefrequency deletion represent Yaxes inblue. are cases (NM) metastatic for non- frequencies Corresponding inmetastatic (red). probe (M)cases sets figureSupplementary 1: 100 100 20 40 60 80 20 40 60 80 0 0 chr14 1 chr14 88 chr14 175 chr14 262 chr9 -MTAP 349 chr9 -MTAP 436 523 chr9-CDKN2A 610 Histogram presenting the 2000 more frequently deleted deleted frequently the 2000 more presenting Histogram chr9-CDKN2A 697 chr9-CDKN2A 784 chr9-CDKN2B 871 chr9-CDKN2B 958 chr9-CDKN2B 1045 chr22 1132 chr22 1219 chr22 1306 chr22 1393 1480 1567 M NM 1654 1741 1828 1915 M NM GIST14 GIST2 GIST16 GIST3 GIST19 GIST63 GIST9 GIST38 GIST61 GIST39 GIST56 GIST37 GIST47 GIST58 GIST28 GIST5 GIST17 GIST57 GIST47 GIST58 GIST28 GIST5 GIST17 GIST57 CDKN2A Supplementary figure 2: Chromosome 9 genomic profiles of the 18 metastatic GISTs (upper panel). Deletions and gains are indicated in green and red, respectively; and color intensity is proportional to copy number changes. A detailed view is given (bottom panel) for the 6 cases presenting a homozygous 9p21 deletion targeting CDKN2A locus (dark green). -
Oddpols 2021 International Conference on Transcription Mechanism and Regulation Table of Contents: Oddpols 2021 Organizers and Sponsors
OddPols 2021 International Conference on Transcription Mechanism and Regulation Table of Contents: OddPols 2021 Organizers and Sponsors.............................................................................1 Call for Summations and Papers............................................................................................2 Information for Zoom Breakout Rooms and Socialization.....................................................3 OddPols 2021 Virtual Schedule .............................................................................................4 Day 1.............................................................................................................................4 Day 2.............................................................................................................................6 Day 3.............................................................................................................................8 Day 4...........................................................................................................................10 Day 5...........................................................................................................................12 Abstracts..............................................................................................................................14 Participant Directory..........................................................................................................137 Organizing Committee: Craig Cameron, U. of North Carolina Astrid -
ADGRV1 Is Implicated in Myoclonic Epilepsy
Accepted: 20 November 2017 DOI: 10.1111/epi.13980 FULL-LENGTH ORIGINAL RESEARCH ADGRV1 is implicated in myoclonic epilepsy Kenneth A. Myers1 | Steven Nasioulas2 | Amber Boys3 | Jacinta M. McMahon1 | Howard Slater3 | Paul Lockhart2,4 | Desiree du Sart3 | Ingrid E. Scheffer1,2,5,6 1Department of Medicine, Epilepsy Research Centre, University of Summary Melbourne, Austin Health, Heidelberg, Objective: To investigate the significance of variation in ADGRV1 (also known Vic., Australia as GPR98, MASS1, and VLGR1), MEF2C, and other genes at the 5q14.3 chromo- 2Department of Paediatrics, University of somal locus in myoclonic epilepsy. Melbourne, Parkville, Vic., Australia 3 Methods: Victorian Clinical Genetics Services, We studied the epilepsy phenotypes of 4 individuals with 5q14.3 dele- Melbourne, Vic., Australia tion and found that all had myoclonic seizures. We then screened 6 contiguous 4Bruce Lefroy Centre for Genetic Health genes at 5q14.3, MEF2C, CETN3, MBLAC2, POLR3G, LYSMD3, and ADGRV1, Research, Murdoch Childrens Research in a 95-patient cohort with epilepsy and myoclonic seizures. Of these genes, point Institute, Royal Children’s Hospital, Parkville, Vic., Australia mutations in MEF2C cause a phenotype involving seizures and intellectual dis- 5The Florey Institute of Neuroscience and ability. A role for ADGRV1 in epilepsy has been proposed previously, based on a Mental Health, Heidelberg, Vic., recessive mutation in the Frings mouse model of audiogenic seizures, as well as a Australia shared homologous region with another epilepsy gene, LGI1. 6Department of Neurology, Royal Children’s Hospital, Parkville, Vic., Results: Six patients from the myoclonic epilepsy cohort had likely pathogenic Australia ultra-rare ADGRV1 variants, and statistical analysis showed that ultra-rare variants were significantly overrepresented when compared to healthy population data Correspondence Ingrid Scheffer, Epilepsy Research Centre, from the Genome Aggregation Database. -
Powerful Gene-Based Testing by Integrating Long-Range Chromatin Interactions and Knockoff Genotypes
medRxiv preprint doi: https://doi.org/10.1101/2021.07.14.21260405; this version posted July 18, 2021. The copyright holder for this preprint (which was not certified by peer review) is the author/funder, who has granted medRxiv a license to display the preprint in perpetuity. It is made available under a CC-BY-NC-ND 4.0 International license . Powerful gene-based testing by integrating long-range chromatin interactions and knockoff genotypes Shiyang Ma1, James L. Dalgleish1, Justin Lee2, Chen Wang1, Linxi Liu3, Richard Gill4;5, Joseph D. Buxbaum6, Wendy Chung7, Hugues Aschard8, Edwin K. Silverman9, Michael H. Cho9, Zihuai He2;10, Iuliana Ionita-Laza1;# 1 Department of Biostatistics, Columbia University, New York, NY, 10032, USA 2 Quantitative Sciences Unit, Department of Medicine, Stanford University, Stanford, CA, 94305, USA 3 Department of Statistics, University of Pittsburgh, Pittsburgh, PA, 15260, USA 4 Department of Human Genetics, Genentech, South San Francisco, CA, 94080, USA 5 Department of Epidemiology, Columbia University, New York, NY, 10032, USA 6 Departments of Psychiatry, Neuroscience, and Genetics and Genomic Sciences, Icahn School of Medicine at Mount Sinai, New York, NY, 10029, USA 7 Department of Pediatrics and Medicine, Herbert Irving Comprehensive Cancer Center, Columbia Uni- versity Irving Medical Center, New York, NY, 10032, USA 8 Department of Computational Biology, Institut Pasteur, Paris, France 9 Channing Division of Network Medicine and Division of Pulmonary and Critical Care Medicine, Brigham and Women’s Hospital and Harvard Medical School, Boston, MA 02115, USA 10 Department of Neurology and Neurological Sciences, Stanford University, Stanford, CA 94305, USA # e-mail: [email protected] Abstract Gene-based tests are valuable techniques for identifying genetic factors in complex traits.