Identification of RNA-Binding Proteins As Targetable Putative Oncogenes
Total Page:16
File Type:pdf, Size:1020Kb
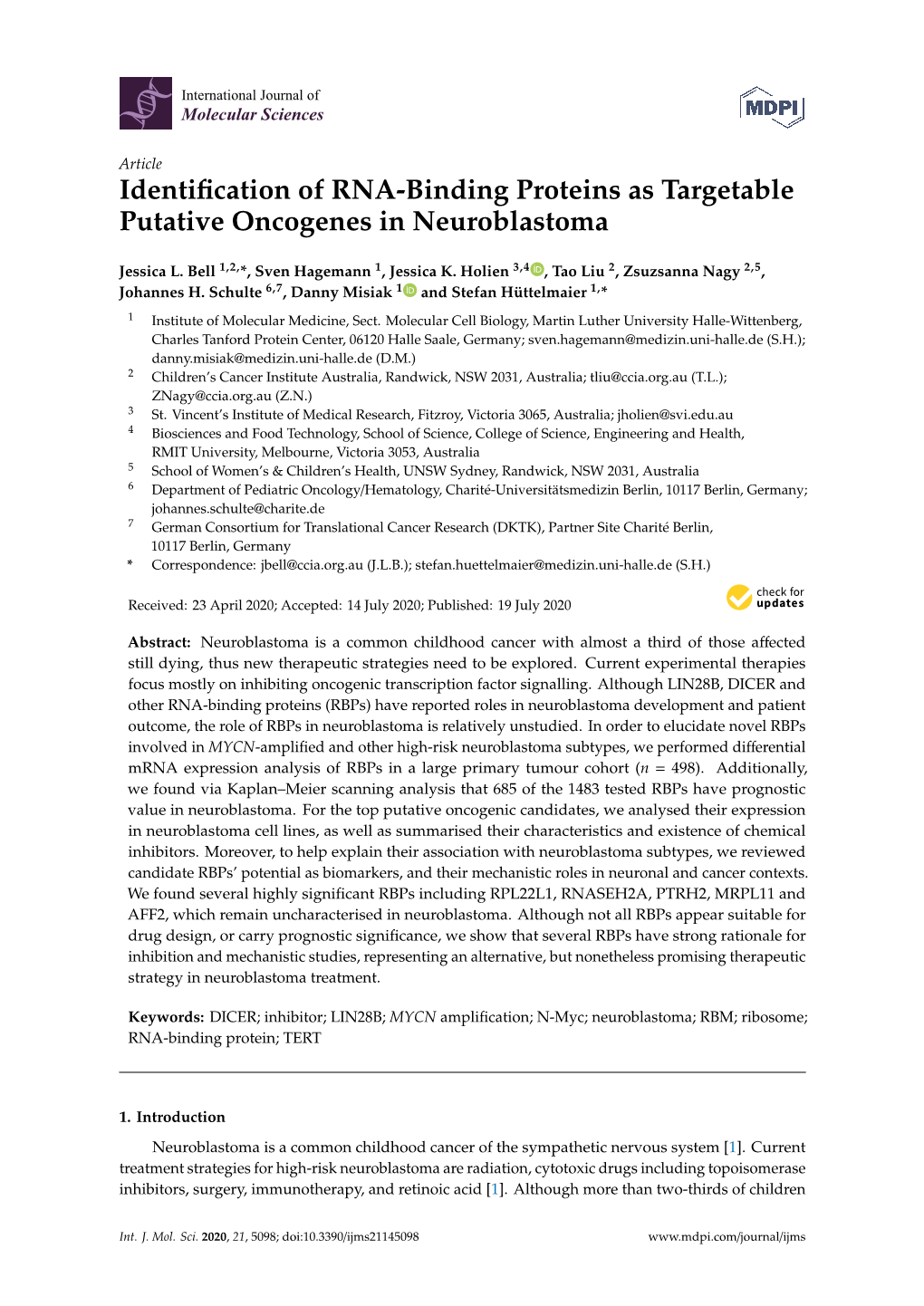
Load more
Recommended publications
-
SRC Antibody - N-Terminal Region (ARP32476 P050) Data Sheet
SRC antibody - N-terminal region (ARP32476_P050) Data Sheet Product Number ARP32476_P050 Product Name SRC antibody - N-terminal region (ARP32476_P050) Size 50ug Gene Symbol SRC Alias Symbols ASV; SRC1; c-SRC; p60-Src Nucleotide Accession# NM_005417 Protein Size (# AA) 536 amino acids Molecular Weight 60kDa Product Format Lyophilized powder NCBI Gene Id 6714 Host Rabbit Clonality Polyclonal Official Gene Full Name V-src sarcoma (Schmidt-Ruppin A-2) viral oncogene homolog (avian) Gene Family SH2D This is a rabbit polyclonal antibody against SRC. It was validated on Western Blot by Aviva Systems Biology. At Aviva Systems Biology we manufacture rabbit polyclonal antibodies on a large scale (200-1000 Description products/month) of high throughput manner. Our antibodies are peptide based and protein family oriented. We usually provide antibodies covering each member of a whole protein family of your interest. We also use our best efforts to provide you antibodies recognize various epitopes of a target protein. For availability of antibody needed for your experiment, please inquire (). Peptide Sequence Synthetic peptide located within the following region: QTPSKPASADGHRGPSAAFAPAAAEPKLFGGFNSSDTVTSPQRAGPLAGG This gene is highly similar to the v-src gene of Rous sarcoma virus. This proto-oncogene may play a role in the Description of Target regulation of embryonic development and cell growth. SRC protein is a tyrosine-protein kinase whose activity can be inhibited by phosphorylation by c-SRC kinase. Mutations in this gene could be involved in the -
Topological Scoring of Protein Interaction Networks
bioRxiv preprint doi: https://doi.org/10.1101/438408; this version posted October 8, 2018. The copyright holder for this preprint (which was not certified by peer review) is the author/funder. All rights reserved. No reuse allowed without permission. Topological Scoring of Protein Interaction Networks Mihaela E. Sardiu1, Joshua M. Gilmore1,2, Brad D. Groppe1,3, Arnob Dutta1,4, Laurence Florens1, and Michael P. Washburn1,5‡ 1Stowers Institute for Medical Research, Kansas City, MO 64110 U.S.A. 2Current Address: Boehringer Ingelheim Vetmedica, St. Joseph, MO 64506 U.S.A. 3Current Address: Thermo Fisher Scientific, Waltham, MA 02451, U.S.A. 4Current Address: Department of Cell and Molecular Biology, University of Rhode Island, 287 CBLS, 120 Flagg Road, Kingston, RI 02881. 5 Department of Pathology and Laboratory Medicine, The University of Kansas Medical Center, 3901 Rainbow Boulevard, Kansas City, Kansas 66160, USA ‡To whom correspondence should be addressed: Michael Washburn, Ph.D. Stowers Institute for Medical Research 1000 E. 50th St. Kansas City, MO 64110 Phone: 816-926-4457 E-mail: [email protected] 1 bioRxiv preprint doi: https://doi.org/10.1101/438408; this version posted October 8, 2018. The copyright holder for this preprint (which was not certified by peer review) is the author/funder. All rights reserved. No reuse allowed without permission. Abstract It remains a significant challenge to define individual protein associations within networks where an individual protein can directly interact with other proteins and/or be part of large complexes, which contain functional modules. Here we demonstrate the topological scoring (TopS) algorithm for the analysis of quantitative proteomic analyses of affinity purifications. -
Analysis of Gene Expression Data for Gene Ontology
ANALYSIS OF GENE EXPRESSION DATA FOR GENE ONTOLOGY BASED PROTEIN FUNCTION PREDICTION A Thesis Presented to The Graduate Faculty of The University of Akron In Partial Fulfillment of the Requirements for the Degree Master of Science Robert Daniel Macholan May 2011 ANALYSIS OF GENE EXPRESSION DATA FOR GENE ONTOLOGY BASED PROTEIN FUNCTION PREDICTION Robert Daniel Macholan Thesis Approved: Accepted: _______________________________ _______________________________ Advisor Department Chair Dr. Zhong-Hui Duan Dr. Chien-Chung Chan _______________________________ _______________________________ Committee Member Dean of the College Dr. Chien-Chung Chan Dr. Chand K. Midha _______________________________ _______________________________ Committee Member Dean of the Graduate School Dr. Yingcai Xiao Dr. George R. Newkome _______________________________ Date ii ABSTRACT A tremendous increase in genomic data has encouraged biologists to turn to bioinformatics in order to assist in its interpretation and processing. One of the present challenges that need to be overcome in order to understand this data more completely is the development of a reliable method to accurately predict the function of a protein from its genomic information. This study focuses on developing an effective algorithm for protein function prediction. The algorithm is based on proteins that have similar expression patterns. The similarity of the expression data is determined using a novel measure, the slope matrix. The slope matrix introduces a normalized method for the comparison of expression levels throughout a proteome. The algorithm is tested using real microarray gene expression data. Their functions are characterized using gene ontology annotations. The results of the case study indicate the protein function prediction algorithm developed is comparable to the prediction algorithms that are based on the annotations of homologous proteins. -
Splicing Regulatory Factors in Breast Cancer Hallmarks and Disease Progression
www.oncotarget.com Oncotarget, 2019, Vol. 10, (No. 57), pp: 6021-6037 Review Splicing regulatory factors in breast cancer hallmarks and disease progression Esmee Koedoot1, Liesanne Wolters1, Bob van de Water1 and Sylvia E. Le Dévédec1 1Division of Drug Discovery and Safety, LACDR, Leiden University, Leiden, The Netherlands Correspondence to: Sylvia E. Le Dévédec, email: [email protected] Keywords: hallmarks of cancer; breast cancer; alternative splicing; splice factors; RNA sequencing Received: April 23, 2019 Accepted: August 29, 2019 Published: October 15, 2019 Copyright: Koedoot et al. This is an open-access article distributed under the terms of the Creative Commons Attribution License 3.0 (CC BY 3.0), which permits unrestricted use, distribution, and reproduction in any medium, provided the original author and source are credited. ABSTRACT By regulating transcript isoform expression levels, alternative splicing provides an additional layer of protein control. Recent studies show evidence that cancer cells use different splicing events to fulfill their requirements in order to develop, progress and metastasize. However, there has been less attention for the role of the complex catalyzing the complicated multistep splicing reaction: the spliceosome. The spliceosome consists of multiple sub-complexes in total comprising 244 proteins or splice factors and 5 associated RNA molecules. Here we discuss the role of splice factors in the oncogenic processes tumors cells need to fulfill their oncogenic properties (the so-called the hallmarks of cancer). Despite the fact that splice factors have been investigated only recently, they seem to play a prominent role in already five hallmarks of cancer: angiogenesis, resisting cell death, sustaining proliferation, deregulating cellular energetics and invasion and metastasis formation by affecting major signaling pathways such as epithelial-to-mesenchymal transition, the Warburg effect, DNA damage response and hormone receptor dependent proliferation. -
Allele-Specific Expression of Ribosomal Protein Genes in Interspecific Hybrid Catfish
Allele-specific Expression of Ribosomal Protein Genes in Interspecific Hybrid Catfish by Ailu Chen A dissertation submitted to the Graduate Faculty of Auburn University in partial fulfillment of the requirements for the Degree of Doctor of Philosophy Auburn, Alabama August 1, 2015 Keywords: catfish, interspecific hybrids, allele-specific expression, ribosomal protein Copyright 2015 by Ailu Chen Approved by Zhanjiang Liu, Chair, Professor, School of Fisheries, Aquaculture and Aquatic Sciences Nannan Liu, Professor, Entomology and Plant Pathology Eric Peatman, Associate Professor, School of Fisheries, Aquaculture and Aquatic Sciences Aaron M. Rashotte, Associate Professor, Biological Sciences Abstract Interspecific hybridization results in a vast reservoir of allelic variations, which may potentially contribute to phenotypical enhancement in the hybrids. Whether the allelic variations are related to the downstream phenotypic differences of interspecific hybrid is still an open question. The recently developed genome-wide allele-specific approaches that harness high- throughput sequencing technology allow direct quantification of allelic variations and gene expression patterns. In this work, I investigated allele-specific expression (ASE) pattern using RNA-Seq datasets generated from interspecific catfish hybrids. The objective of the study is to determine the ASE genes and pathways in which they are involved. Specifically, my study investigated ASE-SNPs, ASE-genes, parent-of-origins of ASE allele and how ASE would possibly contribute to heterosis. My data showed that ASE was operating in the interspecific catfish system. Of the 66,251 and 177,841 SNPs identified from the datasets of the liver and gill, 5,420 (8.2%) and 13,390 (7.5%) SNPs were identified as significant ASE-SNPs, respectively. -
Molecular Basis for the Distinct Cellular Functions of the Lsm1-7 and Lsm2-8 Complexes
bioRxiv preprint doi: https://doi.org/10.1101/2020.04.22.055376; this version posted April 23, 2020. The copyright holder for this preprint (which was not certified by peer review) is the author/funder, who has granted bioRxiv a license to display the preprint in perpetuity. It is made available under aCC-BY-NC-ND 4.0 International license. Molecular basis for the distinct cellular functions of the Lsm1-7 and Lsm2-8 complexes Eric J. Montemayor1,2, Johanna M. Virta1, Samuel M. Hayes1, Yuichiro Nomura1, David A. Brow2, Samuel E. Butcher1 1Department of Biochemistry, University of Wisconsin-Madison, Madison, WI, USA. 2Department of Biomolecular Chemistry, University of Wisconsin School of Medicine and Public Health, Madison, WI, USA. Correspondence should be addressed to E.J.M. ([email protected]) and S.E.B. ([email protected]). Abstract Eukaryotes possess eight highly conserved Lsm (like Sm) proteins that assemble into circular, heteroheptameric complexes, bind RNA, and direct a diverse range of biological processes. Among the many essential functions of Lsm proteins, the cytoplasmic Lsm1-7 complex initiates mRNA decay, while the nuclear Lsm2-8 complex acts as a chaperone for U6 spliceosomal RNA. It has been unclear how these complexes perform their distinct functions while differing by only one out of seven subunits. Here, we elucidate the molecular basis for Lsm-RNA recognition and present four high-resolution structures of Lsm complexes bound to RNAs. The structures of Lsm2-8 bound to RNA identify the unique 2′,3′ cyclic phosphate end of U6 as a prime determinant of specificity. In contrast, the Lsm1-7 complex strongly discriminates against cyclic phosphates and tightly binds to oligouridylate tracts with terminal purines. -
Distinct Basket Nucleoporins Roles in Nuclear Pore Function and Gene Expression
bioRxiv preprint doi: https://doi.org/10.1101/685263; this version posted June 28, 2019. The copyright holder for this preprint (which was not certified by peer review) is the author/funder. This article is a US Government work. It is not subject to copyright under 17 USC 105 and is also made available for use under a CC0 license. Distinct Basket Nucleoporins roles in Nuclear Pore Function and Gene Expression: Tpr is an integral component of the TREX-2 mRNA export pathway Vasilisa Aksenova1, Hang Noh Lee1, †, Alexandra Smith1, †, Shane Chen1, †, Prasanna Bhat3, †, James Iben2, Carlos Echeverria1, Beatriz Fontoura3, Alexei Arnaoutov1 and Mary Dasso1, * 1Division of Molecular and Cellular Biology, National Institute of Child Health and Human Development, National Institutes of Health, Bethesda, MD 20892, USA. 2Molecular Genomics Core, National Institute of Child Health and Human Development, National Institutes of Health, Bethesda, Maryland 20879 3Department of Cell Biology, University of Texas Southwestern Medical Center, Dallas, TX 75390, USA. † These authors contributed equally to this work. *Correspondence: [email protected]. Acronyms: NPC – nuclear pore complex; BSK-NUPs – basket nucleoporins; NG – NeonGreen; AID - Auxin Inducible Degron 1 bioRxiv preprint doi: https://doi.org/10.1101/685263; this version posted June 28, 2019. The copyright holder for this preprint (which was not certified by peer review) is the author/funder. This article is a US Government work. It is not subject to copyright under 17 USC 105 and is also made available for use under a CC0 license. Abstract Nuclear pore complexes (NPCs) are important for many processes beyond nucleocytoplasmic trafficking, including protein modification, chromatin remodeling, transcription, mRNA processing and mRNA export. -
A Computational Approach for Defining a Signature of Β-Cell Golgi Stress in Diabetes Mellitus
Page 1 of 781 Diabetes A Computational Approach for Defining a Signature of β-Cell Golgi Stress in Diabetes Mellitus Robert N. Bone1,6,7, Olufunmilola Oyebamiji2, Sayali Talware2, Sharmila Selvaraj2, Preethi Krishnan3,6, Farooq Syed1,6,7, Huanmei Wu2, Carmella Evans-Molina 1,3,4,5,6,7,8* Departments of 1Pediatrics, 3Medicine, 4Anatomy, Cell Biology & Physiology, 5Biochemistry & Molecular Biology, the 6Center for Diabetes & Metabolic Diseases, and the 7Herman B. Wells Center for Pediatric Research, Indiana University School of Medicine, Indianapolis, IN 46202; 2Department of BioHealth Informatics, Indiana University-Purdue University Indianapolis, Indianapolis, IN, 46202; 8Roudebush VA Medical Center, Indianapolis, IN 46202. *Corresponding Author(s): Carmella Evans-Molina, MD, PhD ([email protected]) Indiana University School of Medicine, 635 Barnhill Drive, MS 2031A, Indianapolis, IN 46202, Telephone: (317) 274-4145, Fax (317) 274-4107 Running Title: Golgi Stress Response in Diabetes Word Count: 4358 Number of Figures: 6 Keywords: Golgi apparatus stress, Islets, β cell, Type 1 diabetes, Type 2 diabetes 1 Diabetes Publish Ahead of Print, published online August 20, 2020 Diabetes Page 2 of 781 ABSTRACT The Golgi apparatus (GA) is an important site of insulin processing and granule maturation, but whether GA organelle dysfunction and GA stress are present in the diabetic β-cell has not been tested. We utilized an informatics-based approach to develop a transcriptional signature of β-cell GA stress using existing RNA sequencing and microarray datasets generated using human islets from donors with diabetes and islets where type 1(T1D) and type 2 diabetes (T2D) had been modeled ex vivo. To narrow our results to GA-specific genes, we applied a filter set of 1,030 genes accepted as GA associated. -
Bppart and Bpmax: RNA-RNA Interaction Partition Function and Structure Prediction for the Base Pair Counting Model
BPPart and BPMax: RNA-RNA Interaction Partition Function and Structure Prediction for the Base Pair Counting Model Ali Ebrahimpour-Boroojeny, Sanjay Rajopadhye, and Hamidreza Chitsaz ∗ Department of Computer Science, Colorado State University Abstract A few elite classes of RNA-RNA interaction (RRI), with complex roles in cellular functions such as miRNA-target and lncRNAs in human health, have already been studied. Accordingly, RRI bioinfor- matics tools tailored for those elite classes have been proposed in the last decade. Interestingly, there are somewhat unnoticed mRNA-mRNA interactions in the literature with potentially drastic biological roles. Hence, there is a need for high-throughput generic RRI bioinformatics tools. We revisit our RRI partition function algorithm, piRNA, which happens to be the most comprehensive and computationally-intensive thermodynamic model for RRI. We propose simpler models that are shown to retain the vast majority of the thermodynamic information that piRNA captures. We simplify the energy model and instead consider only weighted base pair counting to obtain BPPart for Base-pair Partition function and BPMax for Base-pair Maximization which are 225 and 1350 faster ◦ × × than piRNA, with a correlation of 0.855 and 0.836 with piRNA at 37 C on 50,500 experimentally charac- terized RRIs. This correlation increases to 0.920 and 0.904, respectively, at 180◦C. − Finally, we apply our algorithm BPPart to discover two disease-related RNAs, SNORD3D and TRAF3, and hypothesize their potential roles in Parkinson's disease and Cerebral Autosomal Dominant Arteri- opathy with Subcortical Infarcts and Leukoencephalopathy (CADASIL). 1 Introduction Since mid 1990s with the advent of RNA interference discovery, RNA-RNA interaction (RRI) has moved to the spotlight in modern, post-genome biology. -
An Adipocyte-Specific Lncrap2 - Igf2bp2 Complex Enhances Adipogenesis and Energy Expenditure by Stabilizing Target Mrnas
bioRxiv preprint doi: https://doi.org/10.1101/2020.09.29.318980; this version posted September 29, 2020. The copyright holder for this preprint (which was not certified by peer review) is the author/funder, who has granted bioRxiv a license to display the preprint in perpetuity. It is made available under aCC-BY-NC-ND 4.0 International license. An adipocyte-specific lncRAP2 - Igf2bp2 complex enhances adipogenesis and energy expenditure by stabilizing target mRNAs Juan R. Alvarez-Dominguez1,4, Sally Winther2, Jacob B. Hansen2, Harvey F. Lodish1,3,* and Marko Knoll1,5,* 1 Whitehead Institute for Biomedical Research, 455 Main Street, Cambridge, MA 02142, USA 2 Department of Biology, University of Copenhagen, Universitetsparken 13, DK-2100 Copenhagen, Denmark 3 Departments of Biology and Biological Engineering, Massachusetts Institute of Technology, 21 Ames Street, Cambridge, MA, 02142, USA 4 Department of Stem Cell and Regenerative Biology, Harvard Stem Cell Institute, Harvard University, Cambridge, MA 02138, USA 5 Institute for Diabetes Research, Helmholtz Zentrum München, Heidemannstrasse 1, 80939 München, Germany *correspondence: [email protected], [email protected] bioRxiv preprint doi: https://doi.org/10.1101/2020.09.29.318980; this version posted September 29, 2020. The copyright holder for this preprint (which was not certified by peer review) is the author/funder, who has granted bioRxiv a license to display the preprint in perpetuity. It is made available under aCC-BY-NC-ND 4.0 International license. Abstract lncRAP2 is a conserved cytoplasmic adipocyte-specific lncRNA required for adipogenesis. Using hybridization-based purification combined with in vivo interactome analyses, we show that lncRAP2 forms ribonucleoprotein complexes with several mRNA stability and translation modulators, among them Igf2bp2. -
Essential Genes and Their Role in Autism Spectrum Disorder
University of Pennsylvania ScholarlyCommons Publicly Accessible Penn Dissertations 2017 Essential Genes And Their Role In Autism Spectrum Disorder Xiao Ji University of Pennsylvania, [email protected] Follow this and additional works at: https://repository.upenn.edu/edissertations Part of the Bioinformatics Commons, and the Genetics Commons Recommended Citation Ji, Xiao, "Essential Genes And Their Role In Autism Spectrum Disorder" (2017). Publicly Accessible Penn Dissertations. 2369. https://repository.upenn.edu/edissertations/2369 This paper is posted at ScholarlyCommons. https://repository.upenn.edu/edissertations/2369 For more information, please contact [email protected]. Essential Genes And Their Role In Autism Spectrum Disorder Abstract Essential genes (EGs) play central roles in fundamental cellular processes and are required for the survival of an organism. EGs are enriched for human disease genes and are under strong purifying selection. This intolerance to deleterious mutations, commonly observed haploinsufficiency and the importance of EGs in pre- and postnatal development suggests a possible cumulative effect of deleterious variants in EGs on complex neurodevelopmental disorders. Autism spectrum disorder (ASD) is a heterogeneous, highly heritable neurodevelopmental syndrome characterized by impaired social interaction, communication and repetitive behavior. More and more genetic evidence points to a polygenic model of ASD and it is estimated that hundreds of genes contribute to ASD. The central question addressed in this dissertation is whether genes with a strong effect on survival and fitness (i.e. EGs) play a specific oler in ASD risk. I compiled a comprehensive catalog of 3,915 mammalian EGs by combining human orthologs of lethal genes in knockout mice and genes responsible for cell-based essentiality. -
Investigation of the Underlying Hub Genes and Molexular Pathogensis in Gastric Cancer by Integrated Bioinformatic Analyses
bioRxiv preprint doi: https://doi.org/10.1101/2020.12.20.423656; this version posted December 22, 2020. The copyright holder for this preprint (which was not certified by peer review) is the author/funder. All rights reserved. No reuse allowed without permission. Investigation of the underlying hub genes and molexular pathogensis in gastric cancer by integrated bioinformatic analyses Basavaraj Vastrad1, Chanabasayya Vastrad*2 1. Department of Biochemistry, Basaveshwar College of Pharmacy, Gadag, Karnataka 582103, India. 2. Biostatistics and Bioinformatics, Chanabasava Nilaya, Bharthinagar, Dharwad 580001, Karanataka, India. * Chanabasayya Vastrad [email protected] Ph: +919480073398 Chanabasava Nilaya, Bharthinagar, Dharwad 580001 , Karanataka, India bioRxiv preprint doi: https://doi.org/10.1101/2020.12.20.423656; this version posted December 22, 2020. The copyright holder for this preprint (which was not certified by peer review) is the author/funder. All rights reserved. No reuse allowed without permission. Abstract The high mortality rate of gastric cancer (GC) is in part due to the absence of initial disclosure of its biomarkers. The recognition of important genes associated in GC is therefore recommended to advance clinical prognosis, diagnosis and and treatment outcomes. The current investigation used the microarray dataset GSE113255 RNA seq data from the Gene Expression Omnibus database to diagnose differentially expressed genes (DEGs). Pathway and gene ontology enrichment analyses were performed, and a proteinprotein interaction network, modules, target genes - miRNA regulatory network and target genes - TF regulatory network were constructed and analyzed. Finally, validation of hub genes was performed. The 1008 DEGs identified consisted of 505 up regulated genes and 503 down regulated genes.