Biodegradation of Bacillus Anthracis Endospores in Compost
Total Page:16
File Type:pdf, Size:1020Kb
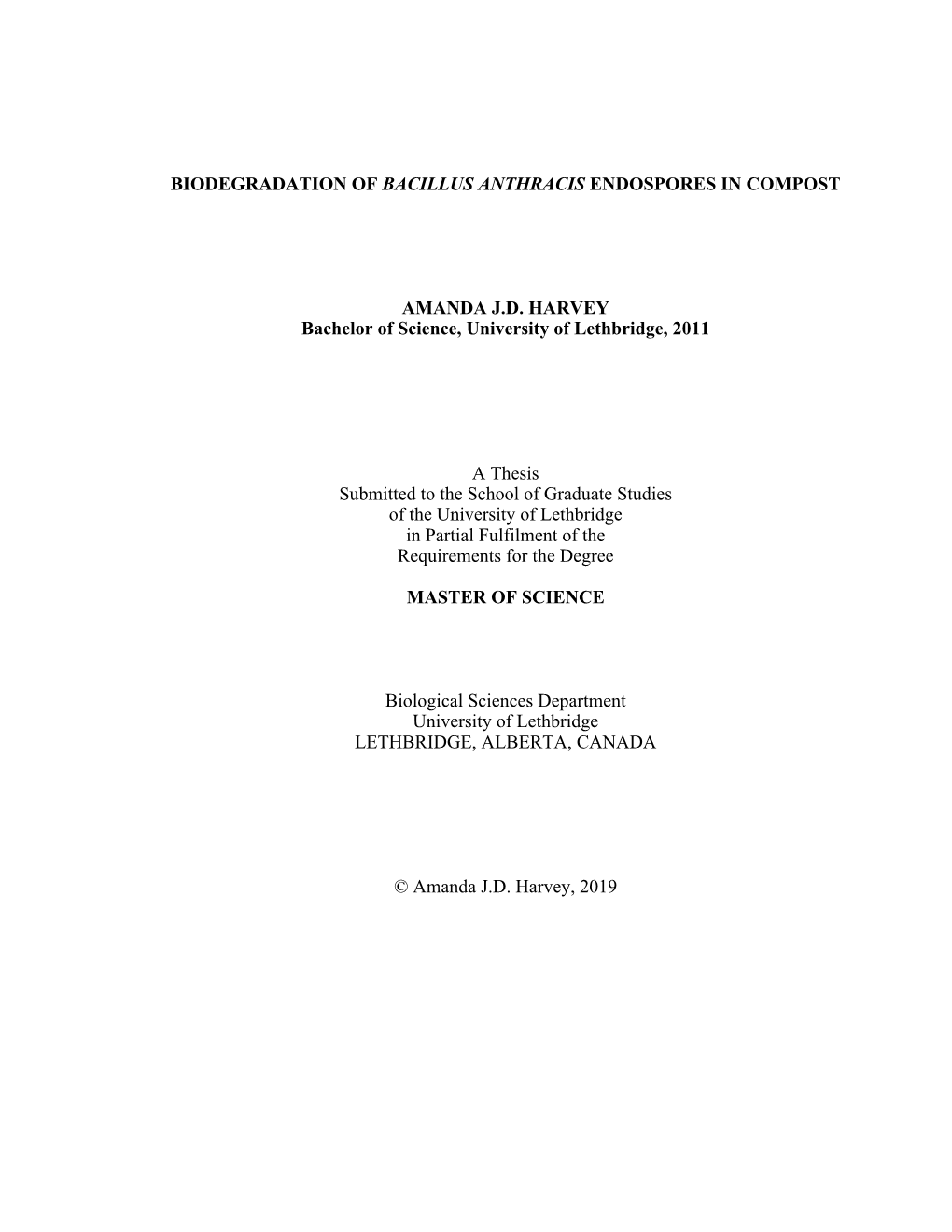
Load more
Recommended publications
-
B. Fragilis Is Mediated by Capsular
bioRxiv preprint doi: https://doi.org/10.1101/2020.08.19.258442; this version posted August 21, 2020. The copyright holder for this preprint (which was not certified by peer review) is the author/funder, who has granted bioRxiv a license to display the preprint in perpetuity. It is made available under aCC-BY-NC-ND 4.0 International license. 1 Hemagglutination by B. fragilis is mediated by capsular 2 polysaccharides and is influenced by host ABO blood type. 3 Kathleen L. Arnolds a, Nancy Moreno-Huizar b, Maggie A. Stanislawski c, 4 Brent Palmer c, Catherine Lozupone c* 5 a Department of Microbiology, University of Colorado Anschutz Medical Campus, 6 Aurora, CO, USA [email protected] 7 b Department of Computer Science, University of Colorado Denver, Denver, CO, USA. 8 c Department of Medicine, University of Colorado Anschutz Medical Campus, Aurora, 9 CO, USA [email protected] 10 11 12 13 14 15 1 bioRxiv preprint doi: https://doi.org/10.1101/2020.08.19.258442; this version posted August 21, 2020. The copyright holder for this preprint (which was not certified by peer review) is the author/funder, who has granted bioRxiv a license to display the preprint in perpetuity. It is made available under aCC-BY-NC-ND 4.0 International license. 16 Hemagglutination by B. fragilis is mediated by capsular polysaccharides and is 17 influenced by host ABO blood type. 18 19 Bacterial hemagglutination of red blood cells (RBCs) is mediated by 20 interactions between bacterial cell components and RBC envelope glycans 21 that vary across individuals by ABO blood type. -
Bacteriophages Targeting Acinetobacter Baumannii Capsule
bioRxiv preprint doi: https://doi.org/10.1101/2020.02.25.965590; this version posted February 26, 2020. The copyright holder for this preprint (which was not certified by peer review) is the author/funder, who has granted bioRxiv a license to display the preprint in perpetuity. It is made available under aCC-BY-NC-ND 4.0 International license. 1 Bacteriophages targeting Acinetobacter baumannii capsule 2 induce antimicrobial resensitization 3 4 Fernando Gordillo Altamirano1*, John H. Forsyth1, Ruzeen Patwa1, Xenia Kostoulias2, Michael Trim1, Dinesh 5 Subedi1, Stuart Archer3, Faye C. Morris2, Cody Oliveira1, Luisa Kielty1, Denis Korneev1, Moira K. O’Bryan1, 6 Trevor J. Lithgow2, Anton Y. Peleg2,4, Jeremy J. Barr1* 7 8 1 School of Biological Sciences, Monash University 9 2 Biomedicine Discovery Institute and Department of Microbiology, Monash University 10 3 Monash Bioinformatics Platform, Faculty of Medicine, Nursing and Health Sciences, Monash University 11 4 Department of Infectious Diseases, The Alfred Hospital and Central Clinical School, Monash University 12 13 *Corresponding authors 14 Fernando Gordillo Altamirano [email protected] 15 Jeremy J. Barr [email protected] 16 School of Biological Sciences, Monash University 17 25 Rainforest Walk, 18 Clayton, 3800, VIC 19 Australia 20 21 Abstract 22 Carbapenem-resistant Acinetobacter baumannii is responsible for frequent, hard-to-treat and often fatal 23 healthcare-associated infections. Phage therapy, the use of viruses that infect and kill bacteria, is an approach 24 gaining significant clinical interest to combat antibiotic-resistant infections. However, a major limitation is that 25 bacteria can develop resistance against phages. Here, we isolated phages with activity against a panel of A. -
An In-Vitro Investigation to Determine the Neuroinflammatory Response of CNS Cells to Oral Bacteria and Their Virulence Factors
An in-vitro investigation to determine the neuroinflammatory response of CNS cells to oral bacteria and their virulence factors by Rahul Previn A thesis submitted in partial fulfilment for the requirements for the degree of MSc (by Research) at the University of Central Lancashire February 2013 i ACKNOWLEDGEMENTS I would like to thank the University of Central Lancashire, UK for the opportunity to undertake my postgraduate research degree. I wish to thank my Principle Investigator (PI) and Director of studies (D0S), Dean, Prof St John Crean for steering me into an interesting, and a hybrid dental-neurosciences project. His inspirational and expert guidance made the challenges of education seem more manageable. I would also like to thank Dr Peter Robinson, my Research Degrees Tutor (RDT), as without his expert help in getting through the various postgraduate degree hurdles would have been impossible. I would like to express my sincere gratitude to my supervisor, Dr Sim Singhrao for the daily guidance, advice, and patience throughout the practical work of the project. I would also like to thank Miss Sophie Poole, currently a PhD student, for ad-hoc assistance in the lab and for guidance in interpreting row data whenever she was nearby. I would like to acknowledge Prof. M. Curtis for the essential reagents I used to investigate my research question without which, my project would be incomplete. Above all, I would like to express my heartfelt gratitude to my family, especially my mother for her undying love, invaluable moral and financial support and encouragement to do well, during my time away from home. -
Bacterial Size, Shape and Arrangement & Cell Structure And
Lecture 13, 14 and 15: bacterial size, shape and arrangement & Cell structure and components of bacteria and Functional anatomy and reproduction in bacteria Bacterial size, shape and arrangement Bacteria are prokaryotic, unicellular microorganisms, which lack chlorophyll pigments. The cell structure is simpler than that of other organisms as there is no nucleus or membrane bound organelles.Due to the presence of a rigid cell wall, bacteria maintain a definite shape, though they vary as shape, size and structure. When viewed under light microscope, most bacteria appear in variations of three major shapes: the rod (bacillus), the sphere (coccus) and the spiral type (vibrio). In fact, structure of bacteria has two aspects, arrangement and shape. So far as the arrangement is concerned, it may Paired (diplo), Grape-like clusters (staphylo) or Chains (strepto). In shape they may principally be Rods (bacilli), Spheres (cocci), and Spirals (spirillum). Size of Bacterial Cell The average diameter of spherical bacteria is 0.5- 2.0 µm. For rod-shaped or filamentous bacteria, length is 1-10 µm and diameter is 0.25-1 .0 µm. E. coli , a bacillus of about average size is 1.1 to 1.5 µm wide by 2.0 to 6.0 µm long. Spirochaetes occasionally reach 500 µm in length and the cyanobacterium Accepted wisdom is that bacteria are smaller than eukaryotes. But certain cyanobacteria are quite large; Oscillatoria cells are 7 micrometers diameter. The bacterium, Epulosiscium fishelsoni , can be seen with the naked eye (600 mm long by 80 mm in diameter). One group of bacteria, called the Mycoplasmas, have individuals with size much smaller than these dimensions. -
Bacterial Capsular Polysaccharides of Pathogens – a Toolbox for Vaccines and Therapeutics
In: Glycome: The Hidden Code in Biology ISBN: 978-1-53619-377-0 Editor: Dipak K. Banerjee © 2021 Nova Science Publishers, Inc. Chapter 13 BACTERIAL CAPSULAR POLYSACCHARIDES OF PATHOGENS – A TOOLBOX FOR VACCINES AND THERAPEUTICS Vamsee Veeramachineni, PhD, Shonoi A. Ming, PhD, Justine Vionnet, PhD and Willie F. Vann*, PhD Laboratory of Bacterial Polysaccharides, Center for Biologics Evaluation and Research, FDA, Silver Spring, MD, US ABSTRACT The bacterial capsule is a hydrated polysaccharide structure that covers the outermost layer of the cell wall. It is an important virulence factor and acts as armor in shielding the bacteria from a variety of environmental pressures and host immune defenses. Considerable structural diversity exits not only between capsular polysaccharides of different bacterial species, but also within the same species. While most pathogenic bacteria are encapsulated, most encapsulated bacteria are not pathogenic. As a result, understanding the structural and immunological diversity of capsules together with cellular components and machinery involved in capsule biosynthesis is paramount in developing new therapeutics to fight deadly bacterial infections. This chapter presents an overview of the capsular polysaccharide of pathogenic bacteria. This overview includes the structural diversity of capsules among virulent bacteria, the organization of capsule genetic elements, the mechanisms of capsule biosynthesis and transport, along with current technologies employed in the preparation of glycoconjugate vaccines. Keywords: bacterial virulence, capsular polysaccharide, K-antigen, capsule diversity, gram- negative bacteria, gram-positive bacteria, capsular gene organization, capsular biosynthesis, ABC transporter pathway, wzy pathway, synthase pathway, capsule transport, glycoconjugate vaccines, vaccine preparation technologies * Corresponding Author’s Email: [email protected]. -
Along the Path of Bacterial Nonulosonic Acids
Faculty of Science and Technology Along the path of bacterial nonulosonic acids A study of the bio- and in vitro synthesis of sialic acid related compounds — Marie-Josée Haglund Halsør A dissertation for the degree of Philosophiae Doctor – June 2019 Along the path of nonulosonic acids A study of the bio- and in vitro synthesis of sialic acid related compounds Marie-Josée Haglund Halsør A dissertation for the degree of Philosophiae Doctor FACULTY OF SCIENCE AND TECHNOLOGY DEPARTMENT OF CHEMISTRY June 2019 "There is a single light of science and to brighten it anywhere is to brighten it everywhere." - Unsourced, credited to Isaac Asimov. Preface “Why?”, and later “How?”. Those two questions are what led me to research, without doubt. I’ve asked them (aloud or not) every day for as long as I can remember, about practically everything. The other thing is being amazed by Nature. The diversity of every aspect and how it all functions as one, somehow. My favorite as a child were the documentaries by “le Commandant Cousteau” (the sharks!), and my dream was to be an oceanographer. I pursued that dream up until my first year of university, when I discovered biochemistry. I had already grown a liking for chemistry, and it was the only discipline that answered the “biological whys and hows” without going into physics. Biochemistry studies and does, both trying to unravel Nature’s secrets and building its own means to do so. It also uses the knowledge to improve human living conditions, at least in theory. I was sold, and here I am. -
The Molecular Basis of Regulation of Bacterial Capsule Assembly by Wzc
ARTICLE https://doi.org/10.1038/s41467-021-24652-1 OPEN The molecular basis of regulation of bacterial capsule assembly by Wzc Yun Yang1,2,3,8, Jiwei Liu1,2,8, Bradley R. Clarke 4, Laura Seidel4, Jani R. Bolla 5,6, Philip N. Ward 1,2,3, ✉ ✉ Peijun Zhang 2,7, Carol V. Robinson 5,6, Chris Whitfield 4 & James H. Naismith 1,2,3 Bacterial extracellular polysaccharides (EPSs) play critical roles in virulence. Many bacteria assemble EPSs via a multi-protein “Wzx-Wzy” system, involving glycan polymerization at the 1234567890():,; outer face of the cytoplasmic/inner membrane. Gram-negative species couple polymerization with translocation across the periplasm and outer membrane and the master regulator of the system is the tyrosine autokinase, Wzc. This near atomic cryo-EM structure of depho- sphorylated Wzc from E. coli shows an octameric assembly with a large central cavity formed by transmembrane helices. The tyrosine autokinase domain forms the cytoplasm region, while the periplasmic region contains small folded motifs and helical bundles. The helical bundles are essential for function, most likely through interaction with the outer membrane translocon, Wza. Autophosphorylation of the tyrosine-rich C-terminus of Wzc results in disassembly of the octamer into multiply phosphorylated monomers. We propose that the cycling between phosphorylated monomer and dephosphorylated octamer regulates glycan polymerization and translocation. 1 Rosalind Franklin Institute, Harwell Campus, Harwell, UK. 2 Division of Structural Biology, The University of Oxford, Oxford, UK. 3 The Research Complex at Harwell, Harwell Campus, Harwell, UK. 4 Department of Molecular and Cellular Biology, The University of Guelph, Guelph, ON, Canada. -
The PTS Components in Klebsiella Pneumoniae Affect Bacterial Capsular Polysaccharide Production and Macrophage Phagocytosis Resistance
microorganisms Article The PTS Components in Klebsiella pneumoniae Affect Bacterial Capsular Polysaccharide Production and Macrophage Phagocytosis Resistance Novaria Sari Dewi Panjaitan 1,†, Yu-Tze Horng 1,†, Chih-Ching Chien 2, Hung-Chi Yang 3, Ren-In You 1 and Po-Chi Soo 1,* 1 Department of Laboratory Medicine and Biotechnology, College of Medicine, Tzu Chi University, No. 701, Sec. 3, Zhongyang Rd., Hualien 97004, Taiwan; [email protected] (N.S.D.P.); [email protected] (Y.-T.H.); [email protected] (R.-I.Y.) 2 Graduate School of Biotechnology and Bioengineering, Yuan Ze University, Taoyuan 32003, Taiwan; [email protected] 3 Department of Medical Laboratory Science and Biotechnology, Yuanpei University of Medical Technology, Hsinchu 30015, Taiwan; [email protected] * Correspondence: [email protected]; Tel.: +886-3-8565301 (ext. 2347) † Contributed equally to this work. Abstract: Capsular polysaccharide (CPS) is a crucial virulence factor for Klebsiella pneumoniae infection. We demonstrated an association of CPS production with two phosphoenolpyruvate:carbohydrate phosphotransferase systems (PTSs). Deficiency of crr, encoding enzyme IIA of PTS, in K. pneumoniae enhanced the transcriptional activities of galF, wzi and gnd, which are in the cps gene cluster, leading to high CPS production. A crr mutant exhibited a higher survival rate in 1% hydrogen peroxide than Citation: Panjaitan, N.S.D.; Horng, the wild-type. The crr mutant showed less sensitivity to engulfment by macrophage (RAW 264.7) Y.-T.; Chien, C.-C.; Yang, H.-C.; You, R.-I.; Soo, P.-C. The PTS Components than the wild-type by observing the intracellular bacteria using confocal laser scanning microscopy in Klebsiella pneumoniae Affect (CLSM) and by calculating the colony-forming units (CFU) of intracellular bacteria. -
Virulence Factors of Meningitis-Causing Bacteria: Enabling Brain Entry Across the Blood–Brain Barrier
International Journal of Molecular Sciences Review Virulence Factors of Meningitis-Causing Bacteria: Enabling Brain Entry across the Blood–Brain Barrier Rosanna Herold, Horst Schroten and Christian Schwerk * Department of Pediatrics, Pediatric Infectious Diseases, Medical Faculty Mannheim, Heidelberg University, 68167 Mannheim, Germany; [email protected] (R.H.); [email protected] (H.S.) * Correspondence: [email protected]; Tel.: +49-621-383-3466 Received: 26 September 2019; Accepted: 25 October 2019; Published: 29 October 2019 Abstract: Infections of the central nervous system (CNS) are still a major cause of morbidity and mortality worldwide. Traversal of the barriers protecting the brain by pathogens is a prerequisite for the development of meningitis. Bacteria have developed a variety of different strategies to cross these barriers and reach the CNS. To this end, they use a variety of different virulence factors that enable them to attach to and traverse these barriers. These virulence factors mediate adhesion to and invasion into host cells, intracellular survival, induction of host cell signaling and inflammatory response, and affect barrier function. While some of these mechanisms differ, others are shared by multiple pathogens. Further understanding of these processes, with special emphasis on the difference between the blood–brain barrier and the blood–cerebrospinal fluid barrier, as well as virulence factors used by the pathogens, is still needed. Keywords: bacteria; blood–brain barrier; blood–cerebrospinal fluid barrier; meningitis; virulence factor 1. Introduction Bacterial meningitis, as are bacterial encephalitis and meningoencephalitis, is an inflammatory disease of the central nervous system (CNS). It can be diagnosed by the presence of bacteria in the CNS. -
7 Structures of Bacterial Polysaccharides
Transworld Research Network 37/661 (2), Fort P.O., Trivandrum-695 023, Kerala, India Progress in the synthesis of complex carbohydrate chains of plant and microbial polysaccharides, 2009: 181-198 ISBN: 978-81-7895-424-0 Editor: Nikolay E. Nifantiev Structures of bacterial 7 polysaccharides Yuriy A. Knirel N.D. Zelinsky Institute of Organic Chemistry, Russian Academy of Sciences Leninsky Prospekt 47, Moscow 119991, Russia Abstract This chapter is devoted to the composition and structure of various bacterial surface glycopolymers: lipopolysaccharides of Gram-negative bacteria, cell- wall anionic polysaccharides of Gram-positive bacteria, including teichoic and lipoteichoic acids, and mycobacterial lipoglycans. Extracellular polysaccharides, which build a protective capsule, participate in biofilm formation or are excreted as slime, are considered too. The occurrence of both monosaccharides and non-carbohydrate groups as components of the polysaccharide is surveyed. Various structural types of the polysaccharides are discussed, including homopolysaccharides and heteropolysaccharides built up of oligosaccharide or oligosaccharide-phosphate repeating units. Attention is paid to the mode of the attachment of various polysaccharides to the cell surface. Correspondence/Reprint request: Dr. Yuriy A. Knirel, N.D. Zelinsky Institute of Organic Chemistry, Russian Academy of Sciences, Leninsky Prospekt 47, Moscow 119991, Russia. E-mail: [email protected] 182 Yuriy A. Knirel 1. Introduction Glycopolymers are components of the cell envelope of various bacteria. In Gram- negative bacteria, the cell envelope consists of the inner (cytoplasmic) and outer membrane and a rigid peptidoglycan (murein) layer in between. The outer leaflet of the outer membrane consists mainly of lipopolysaccharide (LPS, endotoxin). Gram-positive bacteria lack the outer membrane and have a much thicker peptidoglycan layer. -
Gut Biogeography of the Bacterial Microbiota
REVIEWS MICROBIOME Gut biogeography of the bacterial microbiota Gregory P. Donaldson, S. Melanie Lee and Sarkis K. Mazmanian Abstract | Animals assemble and maintain a diverse but host-specific gut microbial community. In addition to characteristic microbial compositions along the longitudinal axis of the intestines, discrete bacterial communities form in microhabitats, such as the gut lumen, colonic mucus layers and colonic crypts. In this Review, we examine how the spatial distribution of symbiotic bacteria among physical niches in the gut affects the development and maintenance of a resilient microbial ecosystem. We consider novel hypotheses for how nutrient selection, immune activation and other mechanisms control the biogeography of bacteria in the gut, and we discuss the relevance of this spatial heterogeneity to health and disease. Microbiota Humans and other mammals harbour a complex gastro- present in the gut microbiota, such as Escherichia coli and 10 The collection of intestinal microbiota that includes all three domains of Clostridium perfringens . Several species of Bacteroides microorganisms (including life (Archaea, Bacteria and Eukarya). This extraordinary can also utilize fucosylated oligosaccharides as carbon bacteria, viruses, fungi and symbiosis, formed by a series of exposures to environ- sources11, suggesting that their colonization of the gut single-celled eukaryotes) that prebiotic inhabit a particular habitat, mental factors, is initiated on contact with the maternal is aided by the properties of milk. Accordingly, 1 such as an animal. vaginal microbiota during birth . Abrupt changes during children of mothers with non‑functional fucosyltrans- the first year of life follow a pattern that corresponds ferase 2 (FUT2), an enzyme required for the fucosylation Syntrophic interactions to gestational age in both mice2 and humans3, which of milk oligosaccharides, display lower levels of faecal Metabolic relationships in suggests that strong deterministic processes shape the Bifidobacterium spp. -
EXPLORING the ROLE of the MAJOR PILIN Pile in the FUNCTIONS of TYPE IV PILI
Université Paris Descartes École doctorale Frontières du vivant (ED 474) Unité de pathogénèse des Infections Vasculaires – Institut Pasteur NEW INSIGHTS INTO MENINGOCOCCAL PATHOGENESIS: EXPLORING THE ROLE OF THE MAJOR PILIN PilE IN THE FUNCTIONS OF TYPE IV PILI Par Paul Kennouche Thèse de doctorat de Biologie Dirigée par le Dr. Guillaume Duménil Présentée et soutenue publiquement le 14 juin 2018 Devant un jury composé de : Pr. Jeremy Derrick Rapporteur Pr. Han Remaut Rapporteur Dr. Olivera Francetic Examinatrice Dr. Alexandra Walczak Examinatrice Dr. Guillaume Duménil Directeur de thèse À mes formidables grand-mères, À toi Nenès, qui ne m’auras pas vu « gagner le dernier bac ». À toi Manou, c’en est fini de « l’École Nationale Scientifique ». Outline INTRODUCTION 1 1 A HISTORICAL OVERVIEW OF THE DIVERSITY OF PROKARYOTIC APPENDAGES 3 1.1 THE FIRST OBSERVED APPENDAGES ARE ASSEMBLED BY TYPE THREE SECRETION SYSTEMS ............. 3 1.1.1 Flagella: rotating bacterial filaments 4 1.1.1.1 Diversity of flagellar systems 4 1.1.1.2 Functions: motility and more 5 1.1.1.3 Structure and assembly 7 1.1.2 The injectisome: needles assembled by the type three secretion system 8 1.1.2.1 Relationships to the flagellum 8 1.1.2.2 Diversity of injectisomes 8 1.1.2.3 A translocation machine 9 1.1.2.4 Structure and assembly 9 1.2 FIMBRIAE: A CATCH-ALL TERM FOR THIN PROKARYOTIC APPENDAGES......................................... 12 1.2.1 Fimbriae of diderm bacteria need to cross two membranes 12 1.2.1.1 Curli: unique amyloid fibers 12 ¬ Discovery of functional