Ignition and Burn in a Small Magnetized Fuel Target
Total Page:16
File Type:pdf, Size:1020Kb
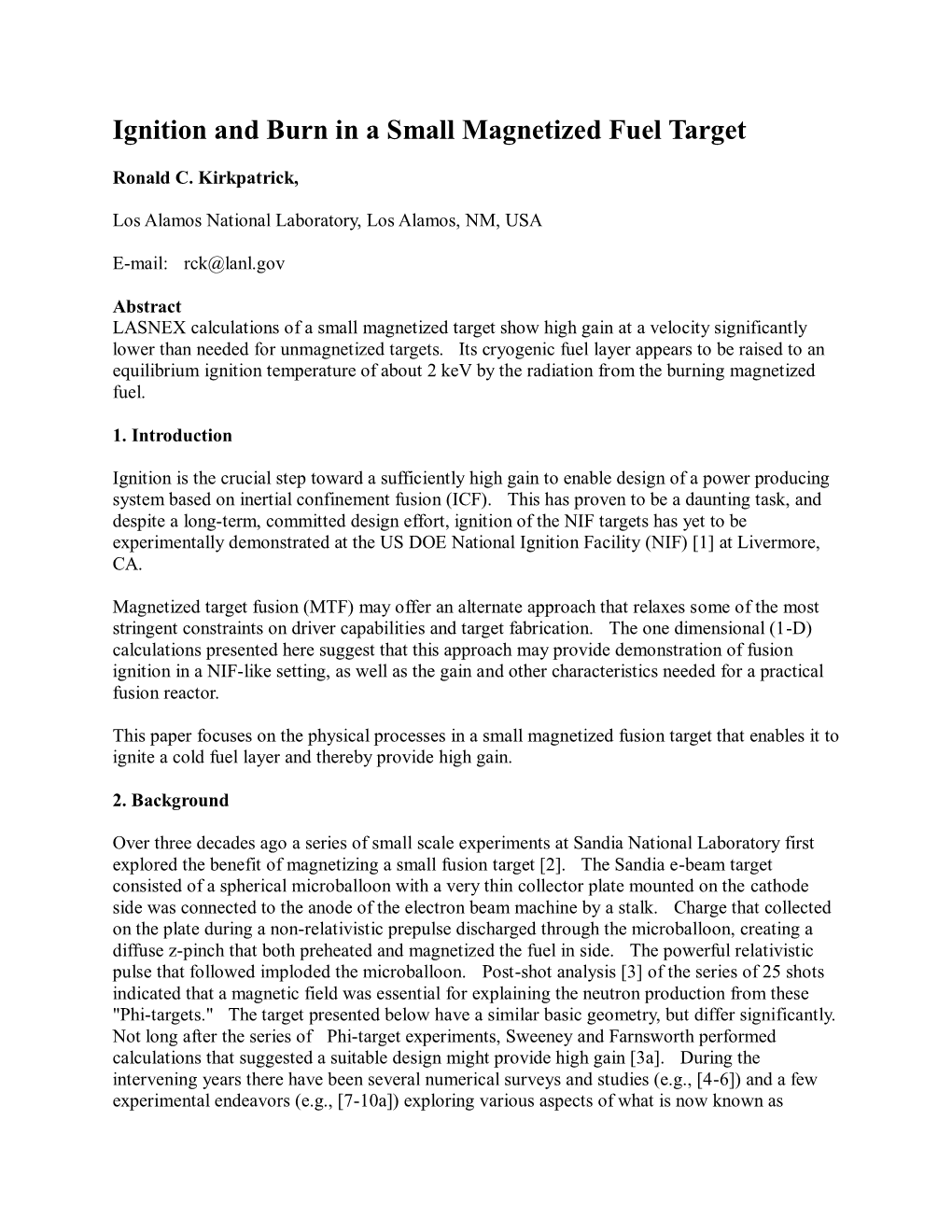
Load more
Recommended publications
-
Reactor Potential for Magnetized Target Fusion
TR.TA-A Report ISSN 1102-2051 VETENSKAP OCH ISRN KTH/ALF/--01/2--SE 1ONST KTH-ALF--01-2 KTH Reactor Potential for Magnetized Target Fusion Jon-Erik Dahlin Research and Training programme on CONTROLLED THERMONUCLEAR FUSION AND PLASMA PHYSICS (Association EURATOM/NFR) FUSION PLASMA PHYSICS ALFV N LABORATORY ROYAL INSTITUTE OF TECHNOLOGY SE-100 44 STOCKHOLM SWEDEN PLEASE BE AWARE THAT ALL OF THE MISSING PAGES IN THIS DOCUMENT WERE ORIGINALLY BLANK TRITA-ALF-2001-02 ISRN KTH/ALF/--01/2--SE Reactor Potential for Magnetized Target Fusion J.-E. Dahlin VETENSKAP OCH KONST Stockholm, June 2001 The Alfven Laboratory Division of Fusion Plasma Physics Royal Institute of Technology SE-100 44 Stockholm, Sweden (Association EURATOM/NFR) Printed by Alfven Laboratory Fusion Plasma Physics Division Royal Institute of Technology SE-100 44 Stockholm Abstract Magnetized Target Fusion (MTF) is a possible pathway to thermonuclear fusion different from both magnetic fusion and inertial confinement fusion. An imploding cylindrical metal liner compresses a preheated and magnetized plasma configuration until thermonuclear conditions are achieved. In this report the Magnetized Target Fusion concept is evaluated and a zero-dimensional computer model of the plasma, liner and circuit as a connected system is designed. The results of running this code are that thermonuclear conditions are achieved indeed, but only during a very short time. At peak compression the pressure from the compressed plasma and mag- netic field is so large reversing the liner implosion into an explosion. The time period of liner motion reversal is termed the dwell time and is crucial to the performance of the fusion system. -
Accelerating Low-Cost Plasma Heating and Assembly – ALPHA
Accelerating Low-Cost Plasma Heating and Assembly – ALPHA PROJECT DESCRIPTIONS California Institute of Technology – Pasadena, CA Prototype Tools to Establish the Viability of the Adiabatic Heating and Compression Mechanisms Required for Magnetized Target Fusion - $800,000 Caltech, in coordination with Los Alamos National Laboratory, will investigate collisions of plasma jets and targets over a wide range of parameters to characterize the scaling of adiabatic heating and compression of liner-driven magnetized target fusion plasmas. The team will propel fast magnetized plasma jets into stationary heavy gases or metal walls. The resulting collision is equivalent to a fast heavy gas or metal liner impacting a stationary magnetized target in a shifted reference frame and allows the non-destructive and rapid investigation of physical phenomena and scaling laws governing the degree of adiabaticity of liner implosions. This study will provide critical information on the interactions and limitations for a variety of possible driver and plasma target combinations being developed across the ALPHA program portfolio. Helion Energy, Inc. – Redmond, WA Staged Magnetic Compression of FRC Targets to Fusion Conditions- $3,971,264 Helion Energy, Inc. will investigate staged magnetic compression of field-reversed configuration (FRC) plasmas, building on past successes to develop a prototype that can attain higher temperatures and fuel density than previously possible. The team will use these results to assess the viability of scaling to a power reactor, which if successful would offer the benefits of simple linear geometry, attractive scaling, and compatibility with modern pulsed power electronics. Lawrence Berkeley National Laboratory – Berkeley, CA MEMS Based Ion Beam Drivers for Magnetized Target Fusion- $2,200,000 Lawrence Berkley National Laboratory (LBNL), in close collaboration with Cornell University, will develop a scalable ion beam driver based on microelectromechanical systems (MEMS) technology. -
Late-Time Simulation of National Ignition Facility Hohlraums
UCRL-JRNL-206693 Late-time simulation of National Ignition Facility Hohlraums D.C. Eder, O.S. Jones, M.M. Marinak, M.T. Tobin, B.J. MacGowan September 21, 2004 Nuclear Fusion 2004 Disclaimer This document was prepared as an account of work sponsored by an agency of the United States Government. Neither the United States Government nor the University of California nor any of their employees, makes any warranty, express or implied, or assumes any legal liability or responsibility for the accuracy, completeness, or usefulness of any information, apparatus, product, or process disclosed, or represents that its use would not infringe privately owned rights. Reference herein to any specific commercial product, process, or service by trade name, trademark, manufacturer, or otherwise, does not necessarily constitute or imply its endorsement, recommendation, or favoring by the United States Government or the University of California. The views and opinions of authors expressed herein do not necessarily state or reflect those of the United States Government or the University of California, and shall not be used for advertising or product endorsement purposes. Late-time simulation of National Ignition Facility hohlraums D. C. Eder, A. E. Koniges, O. S. Jones, M. M. Marinak, M. T. Tobin, and B. J. MacGowan Lawrence Livermore National Laboratory, Livermore, CA 94551 Abstract The late-time (t ≥ 80 ns) behavior of hohlraums designed for the National Ignition Facility (NIF) is simulated using the multiphysics radiation hydrodynamics codes LASNEX and HYDRA. The spatial distribution of x-radiation outside the hohlraum is shown as a function of time. The energy spectrum of the x-ray emission is presented for various hohlraum viewing angles. -
Nuclear Fusion Power – an Overview of History, Present and Future
International Journal of Advanced Network, Monitoring and Controls Volume 04, No.04, 2019 Nuclear Fusion Power – An Overview of History, Present and Future Stewart C. Prager Department of Physics University of Wisconsin – Madison Madison, WI 53706, USA E-mail: [email protected] Summary—Fusion power offers the prospect of an allowing the nuclei to fuse together. Such conditions almost inexhaustible source of energy for future can occur when the temperature increases, causing the generations, but it also presents so far insurmountable ions to move faster and eventually reach speeds high engineering challenges. The fundamental challenge is to enough to bring the ions close enough together. The achieve a rate of heat emitted by a fusion plasma that nuclei can then fuse, causing a release of energy. exceeds the rate of energy injected into the plasma. The main hope is centered on tokamak reactors and II. FUSION TECHNOLOGY stellarators which confine deuterium-tritium plasma In the Sun, massive gravitational forces create the magnetically. right conditions for fusion, but on Earth they are much Keywords-Fusion Energy; Hydrogen Power; Nuclear Fusion harder to achieve. Fusion fuel – different isotopes of hydrogen – must be heated to extreme temperatures of I. INTRODUCTION the order of 50 million degrees Celsius, and must be Today, many countries take part in fusion research kept stable under intense pressure, hence dense enough to some extent, led by the European Union, the USA, and confined for long enough to allow the nuclei to Russia and Japan, with vigorous programs also fuse. The aim of the controlled fusion research underway in China, Brazil, Canada, and Korea. -
Compact Fusion Reactors
Compact fusion reactors Tomas Lind´en Helsinki Institute of Physics 26.03.2015 Fusion research is currently to a large extent focused on tokamak (ITER) and inertial confinement (NIF) research. In addition to these large international or national efforts there are private companies performing fusion research using much smaller devices than ITER or NIF. The attempt to achieve fusion energy production through relatively small and compact devices compared to tokamaks decreases the costs and building time of the reactors and this has allowed some private companies to enter the field, like EMC2, General Fusion, Helion Energy, Lockheed Martin and LPP Fusion. Some of these companies are trying to demonstrate net energy production within the next few years. If they are successful their next step is to attempt to commercialize their technology. In this presentation an overview of compact fusion reactor concepts is given. CERN Colloquium 26th of March 2015 Tomas Lind´en (HIP) Compact fusion reactors 26.03.2015 1 / 37 Contents Contents 1 Introduction 2 Funding of fusion research 3 Basics of fusion 4 The Polywell reactor 5 Lockheed Martin CFR 6 Dense plasma focus 7 MTF 8 Other fusion concepts or companies 9 Summary Tomas Lind´en (HIP) Compact fusion reactors 26.03.2015 2 / 37 Introduction Introduction Climate disruption ! ! Pollution ! ! ! Extinctions Ecosystem Transformation Population growth and consumption There is no silver bullet to solve these issues, but energy production is "#$%&'$($#!)*&+%&+,+!*&!! central to many of these issues. -.$&'.$&$&/!0,1.&$'23+! Economically practical fusion power 4$(%!",55*6'!"2+'%1+!$&! could contribute significantly to meet +' '7%!89 !)%&',62! the future increased energy :&(*61.'$*&!(*6!;*<$#2!-.=%6+! production demands in a sustainable way. -
Emerging Concepts Reactor Subgroup Summary J
LA-UR-99-5178 Emerging Concepts Reactor Subgroup Summary J. Hammer and R. Siemon Emerging Concepts offer unique reactor features, which may lead to a qualitative improvement in cost and maintainability, with associated increased attractiveness to the customer. Table 3 shows some of these unique features grouped by concept: Concept Motivation RFP Low external field; no disruptions Spheromak, FRC Simple geometry; small size; open axial divertor MTF, Flow Pinch Low development cost; compatible with liquid walls Levitated Dipole, Centrifugally confined High β, classical confinement; no current drive Mirrors Low physics risk; linear geometry Electrostatic, IEC, POPS Small unit size; low-cost development; high mass power density; alternate applications Fast Igniter High gain; low recirculating power Table 3: Reactor features of Emerging Concepts. Again, many examples of reactor advantages associated with Emerging Concepts could be given. As one such, there appears at first examination to be a greater accessibility for incorporating liquid walls into many such reactors. This follows from the linear, open geometry of several of the concepts, including FRC, MTF, Flow Pinch, and Mirrors. Organization The reactor subgroup, jointly with the Physics subgroup, heard presentations arranged before the conference on the 11 concepts listed below. These covered a wide range in physical parameter space with radically different reactor embodiments. The reversed field pinch and spheromak talks were held jointly with the Magnetic Confinement sessions. For each concept, a presenter introduced the concept and reviewed progress to date and important physics and reactor issues. The presenter was followed by a reviewer who brought additional insights. Concept Presenter Reviewer RFP S. -
X-Ray Emission from National Ignition Facility Indirect Drive Targets
UCRL-JC-123557 X-Ray Emission from National Ignition Facility Indirect Drive Targets A. T. Anderson, R. A. Managan, M. T. Tobin, and P. F. Peterson AUG 1 6 1338 This paper was prepared for submittal to the American Nuclear Society 12th Topical Meeting on the Technology of Fusion Energy Reno, NV June 16-20,1996 This isapreprintofapaperintendedforpublicationina journal orproceedings. Since changes may be made before publication, this preprint is made available with the understanding that it will not be cited or reproduced without the permission of the author. DISTRIBUTION OF THIS DOCUMENT IS UNIMTED DISCLAIMER This document was prepared as an account of work sponsored by an agency of the United States Government Neither the United States Government nor the University of California nor any of their employees, makes any warranty, express or implied, or assumes any legal liability or responsibility for the accuracy, completeness, or usefulness of any information, apparatus, product, or process disclosed, or represents that its use would not infringe privately owned rights. Reference herein to any specific commercial product, process, or service by trade name, trademark, manufacturer, or otherwise, does not necessarily constitute or imply its-endorsement, recommendation, or favoring by the United States Government or the University of California. The views and opinions of authors expressed herein do not necessarily state or reflect those of the United States Government or the University of California, and shall not be used for advertising or product endorsement purposes. DISCLAIMER Portions of this document may be illegible in electronic image products. Images are produced from the best available origmal document. -
APPENDIX a Why Magnetized Target Fusion Offers a Low-Cost
MTF Proof-of-Principle Proposal App. A. Why MTF offers a low-cost development path. APPENDIX A Why Magnetized Target Fusion Offers A Low-Cost Development Path for Fusion Energy Richard E. Siemon Irvin R. Lindemuth Kurt F. Schoenberg Los Alamos National Laboratory Los Alamos, New Mexico Accepted by Comments on Plasma Physics and Controlled Fusion, December, 1997 1 MTF Proof-of-Principle Proposal App. A. Why MTF offers a low-cost development path. I. Introduction Reasonably priced energy supplies have become an expectation of the developed world and a necessary ingredient for development of Third World countries. The problem of providing large supplies of low-cost energy is a long-term, complex one that requires sustained R&D efforts, in spite of the shadow cast on long-term R&D by the federal deficit problem. The role of fusion energy as a power source was thoroughly reviewed and strongly endorsed in 1995 by the President’s Committee of Advisors on Science and Technology Fusion Review Panel chaired by John Holdren. He argued [Holdren 95]: The options available for meeting the world’s demand for energy in 2050 and beyond are those already in use – fossil fuels, biomass energy, nuclear fission, hydropower, geothermal energy, wind energy, and solar energy – plus, potentially, nuclear fusion. In these circumstances, it should be obvious that there is great merit in the pursuit of diversity in energy options for the next century. There are not so many possibilities altogether. The greater the number of these that can be brought to the point of commercialization, the greater will be the chance that overall energy needs can be met without encountering excessive costs from or unmanageable burdens upon any one source. -
Biographies of Evaluators
BIOGRAPHIES OF EVALUATORS Dr. Edward C. Creutz Dr. Arthur R. Kantrowitz, Chairman Dr. Joseph E. Lannutti Dr. Hans J. Schneider-Muntau Dr. Glenn T. Seaborg Dr. Frederick Seitz Dr. William B. Thompson EDWARD CHESTER CREUTZ Edward Chester Creutz: Education: B.S. Mathematics and Physics, University of Wisconsin, 1936; Ph.D. Physics, U. of Wisconsin, 1939; Thesis: Resonance Scattering of Protons by Lithium. Professional Experience: 1977-1984, Director, Bishop Museum, Honolulu, HI; 197frI977, Acting Deputy Director, National Science Foundation, Washington, DC; 1975--1977, Assistant Director for Mathematical and Physical Sciences, and Engineering, National Science Foundation; 1970-1975, Assistant Director for Research, National Sci ence Foundation (Presidential appointee); 1955-1970, Vice President, Research and De velopment, General Atomic, San Diego, CA; 1955-1956, Scientist at large, Controlled Thermonuclear Program, Atomic Energy Commission, Washington, DC; 1948-1955, Pro fessor and Head, Department of Physics, and Director, Nuclear Research Center, Carnegie Institute of Technology, Pittsburgh, PA; 194fr1948, Associate Professor of Physics, Carnegie Institute of Technology; 1944-1946, Group Leader, Los Alamos, NM; 1942-1944, Group Leader, Manhattan Project, Chicago, IL; 1939-1942, Instructor of Physics, Princeton University, Princeton, NJ. 1945 ff, Consultant to AEC, NASA, Industry. 1960 ff, Editorial Advisory Board: American Nuclear Society, Annual Reviews, Handbuch der Physik, Interdisciplinary Science Reviews, Handbook of Chemistry and Physics. Publications: 65 in fields of Physics, Metallurgy, Mathematics, Botany, and Science Pol icy. Patents: 18 Nuclear Energy Applications. 577 578 Biographies of Evaluaton Honors: Phi Beta Kappa; Tau Beta Pi; Sigma Xi; National Science Foundation Distin guished Service Award; University of Wisconsin, College of Engineering, Distinguished Service Citation; American Nuclear Society, Pioneer Award. -
A Look at US Fusion Research
A Look At US Fusion Research US Fusion Budget: The US fusion budget last year was 951 million dollars [1]. For comparison NASAs’ budget was eight billion [2]. I argue that the current fusion budget is very low and lopsided. It is lopsided because it focuses only on two approaches - which we know will not become commercial. It is low, because this budget gives us no space for anything new. Sadly, this has been the state of things for about the last 20 years or so. Below is a plot of the US fusion budget. Laser fusion (ICF): The US fusion budget gets split in half. Half of the money is sent out to support laser fusion and related technologies. The main goal of that money is to maintain the status quo. It holds up activity at the national labs, a few companies and a few universities. Laser fusion is where you take a small ball of ice and you blast it. The ball of ice is frozen radioactive hydrogen. The beams attack from multiple (60 or 192) directions and squash the fuel [3, 4]. When the beams hit the ice surface, there is an explosion of energy outwards and an equal and opposite compression wave inwards. This compresses the fuel to a temperature and pressure where fusion can occur. For example, a typical temperature could be between 10 and 15 million degrees kelvin and the pressure is 1000x the density of water [3, 4]. A typical implosion would last about 20 nanoseconds. This laser approach to fusion is over sixty years old [5]. -
Compact Fusion Reactors
Compact fusion reactors Tomas Lind´en Helsinki Institute of Physics NST2016, Helsinki, 3rd of November 2016 Fusion research is currently to a large extent focused on tokamak (ITER) and inertial confinement (NIF) research. In addition to these large international or national efforts there are private companies performing fusion research using alternative concepts, that potentially could result on a faster time scale in smaller and cheaper devices than ITER or NIF. The attempt to achieve fusion energy production through relatively small and compact devices compared to standard tokamaks decreases the costs and building time of the reactors and this has allowed several private companies to enter the field, like EMC2, General Fusion, Helion Energy, LPP Fusion, Lockheed Martin, Tokamak Energy and Tri Alpha Energy. These companies are trying to demonstrate the feasibility of their concept. If that is succesfully done, their next step is to try to demonstrate net energy production and after that to attempt to commercialize their technology. In this presentation a very brief overview of compact fusion reactor research is given. Tomas Lind´en (HIP) Compact fusion reactors 03.11.2016 1 / 24 Contents Contents 1 Fusion conditions 2 Plasma confinement 3 The Polywell reactor 4 Lockheed Martin CFR 5 Dense plasma focus 6 MTF 7 Spherical tokamaks 8 Other fusion concepts 9 Summary Tomas Lind´en (HIP) Compact fusion reactors 03.11.2016 2 / 24 Fusion conditions Fusion conditions See Antti Hakolas presentation in this conference on mainline fusion. A useful fusion performance metric is the triple product NτT (1) that has to execeed some threshold value for the fusion reaction in question for the fusion power to exceed radiation and other losses and maintain a constant plasma temperature. -
Nuclear Diagnostics for Inertial Confinement Fusion (ICF) Plasmas
Plasma Physics and Controlled Fusion TOPICAL REVIEW Nuclear diagnostics for Inertial Confinement Fusion (ICF) plasmas To cite this article: J A Frenje 2020 Plasma Phys. Control. Fusion 62 023001 View the article online for updates and enhancements. This content was downloaded from IP address 18.21.213.6 on 07/01/2020 at 20:53 Plasma Physics and Controlled Fusion Plasma Phys. Control. Fusion 62 (2020) 023001 (44pp) https://doi.org/10.1088/1361-6587/ab5137 Topical Review Nuclear diagnostics for Inertial Confinement Fusion (ICF) plasmas J A Frenje Massachusetts Institute of Technology, Cambridge, MA 02139, United States of America E-mail: [email protected] Received 12 December 2017, revised 13 September 2019 Accepted for publication 25 October 2019 Published 7 January 2020 Abstract The field of nuclear diagnostics for Inertial Confinement Fusion (ICF) is broadly reviewed from its beginning in the seventies to present day. During this time, the sophistication of the ICF facilities and the suite of nuclear diagnostics have substantially evolved, generally a consequence of the efforts and experience gained on previous facilities. As the fusion yields have increased several orders of magnitude during these years, the quality of the nuclear-fusion-product measurements has improved significantly, facilitating an increased level of understanding about the physics governing the nuclear phase of an ICF implosion. The field of ICF has now entered an era where the fusion yields are high enough for nuclear measurements to provide spatial, temporal and spectral information, which have proven indispensable to understanding the performance of an ICF implosion. At the same time, the requirements on the nuclear diagnostics have also become more stringent.