Functionalization and Synthesis Of
Total Page:16
File Type:pdf, Size:1020Kb
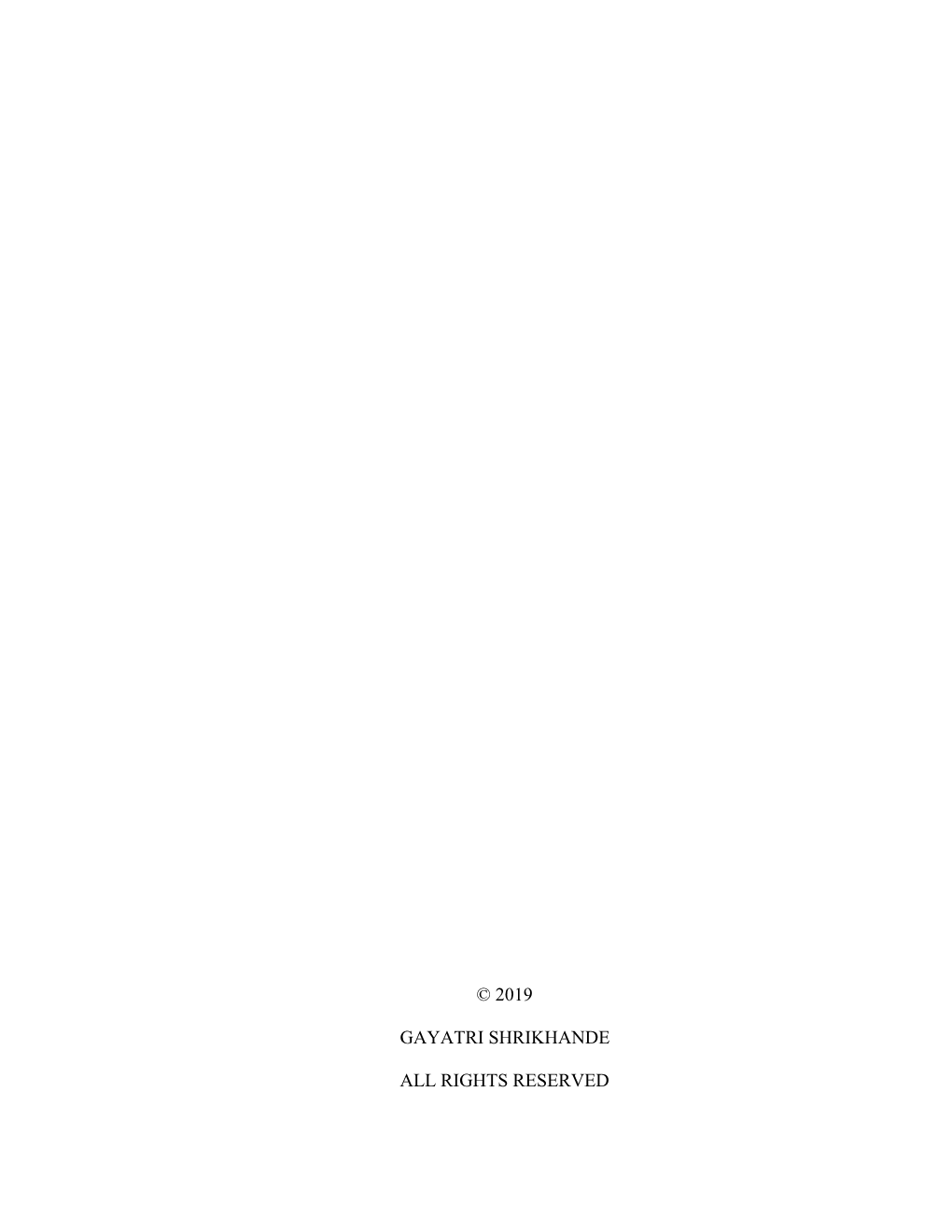
Load more
Recommended publications
-
Packed Column Supercritical Fluid Chromatography: Applications in Environmental Chemistry
Packed Column Supercritical Fluid Chromatography: Applications in Environmental Chemistry Dedication To Steve Örebro Studies in Chemistry 19 NICOLE RIDDELL Packed Column Supercritical Fluid Chromatography: Applications in Environmental Chemistry © Nicole Riddell, 2017 Title: Packed Column Supercritical Fluid Chromatography: Applications in Environmental Chemistry Publisher: Örebro University 2017 www.publications.oru.se Print: Örebro University, Repro 04/2017 ISSN 1651-4270 ISBN 978-91-7529-184-0 Abstract Nicole Riddell (2017): Packed Column Supercritical Fluid Chromatography: Applications in Environmental Chemistry. Örebro Studies in Chemistry 19. Although gas and liquid chromatography have emerged as dominant separation techniques in environmental analytical chemistry, these methods do not allow for the concurrent analysis of chemically diverse groups of persistent organic pollutants (POPs). There are also a small number of compounds which are not easily amenable to either of these traditional separation techniques. The main objective of this thesis was to address these issues by demonstrating the applicability of packed col- umn supercritical fluid chromatography (pSFC) coupled to mass spec- trometry (MS) in various aspects of environmental chemistry. First, pSFC/MS analytical methods were developed for legacy POPs (PCDDs, PCDFs, and PCBs) as well as the emerging environmental con- taminant Dechlorane Plus (DP), and issues relating to the ionization of target analytes when pSFC was coupled to MS were explored. Novel APPI and APCI reagents (fluorobenzene and triethylamine) were opti- mized and real samples (water and soil) were analyzed to demonstrate environmental applicability. The possibility of chiral and preparative scale pSFC separations was then demonstrated through the isolation and characterization of ther- mally labile hexabromocyclododecane (HBCDD) stereoisomers. The analytical pSFC separation of the α-, β-, and γ-HBCDD enantiomers as well as the δ and ε meso forms was shown to be superior to results ob- tained using a published LC method. -
Deuteriodesilylation: a Mild and Selective Method for the Site- Specific Incorporation of Deuterium Into Drug Candidates and Pharmaceutical Structures
DEUTERIODESILYLATION: A MILD AND SELECTIVE METHOD FOR THE SITE- SPECIFIC INCORPORATION OF DEUTERIUM INTO DRUG CANDIDATES AND PHARMACEUTICAL STRUCTURES Kimberly N. Voronin A Thesis Submitted to the University of North Carolina Wilmington in Partial Fulfillment of the Requirements for the Degree of Master of Science Department of Chemistry and Biochemistry University of North Carolina Wilmington 2012 Approved by Advisory Committee Chris V. Galliford Pamela J. Seaton John A. Tyrell Chair Accepted by Dean, Graduate School TABLE OF CONTENTS ABSTRACT ................................................................................................................................... vi ACKNOWLEDGEMENTS .......................................................................................................... vii DEDICATION ............................................................................................................................... ix LIST OF TABLES .......................................................................................................................... x LIST OF FIGURES ...................................................................................................................... xi LIST OF ABBREVIATIONS ...................................................................................................... xiii LIST OF SCHEMES .....................................................................................................................xv CHAPTER 1: INTRODUCTION ....................................................................................................1 -
Interferometric Observations of Warm Deuterated Methanol in the Inner Regions of Low- Mass Protostars
Interferometric observations of warm deuterated methanol in the inner regions of low- mass protostars Taquet, V; Bianchi, E.; Codella, C.; Persson, M., V; Ceccarelli, C.; Cabrit, S.; Jorgensen, J. K.; Kahane, C.; Lopez-Sepulcre, A.; Neri, R. Published in: Astronomy & Astrophysics DOI: 10.1051/0004-6361/201936044 Publication date: 2019 Document version Publisher's PDF, also known as Version of record Document license: CC BY-NC Citation for published version (APA): Taquet, V., Bianchi, E., Codella, C., Persson, M. V., Ceccarelli, C., Cabrit, S., Jorgensen, J. K., Kahane, C., Lopez-Sepulcre, A., & Neri, R. (2019). Interferometric observations of warm deuterated methanol in the inner regions of low-mass protostars. Astronomy & Astrophysics, 632, [A19]. https://doi.org/10.1051/0004- 6361/201936044 Download date: 02. Oct. 2021 A&A 632, A19 (2019) https://doi.org/10.1051/0004-6361/201936044 Astronomy & © ESO 2019 Astrophysics Interferometric observations of warm deuterated methanol in the inner regions of low-mass protostars? V. Taquet1, E. Bianchi2,1, C. Codella1,2, M. V. Persson3, C. Ceccarelli2, S. Cabrit4, J. K. Jørgensen5, C. Kahane2, A. López-Sepulcre2,6, and R. Neri6 1 INAF, Osservatorio Astrofisico di Arcetri, Largo E. Fermi 5, 50125 Firenze, Italy e-mail: [email protected] 2 IPAG, Université Grenoble Alpes, CNRS, 38000 Grenoble, France 3 Department of Space, Earth, and Environment, Chalmers University of Technology, Onsala Space Observatory, 439 92 Onsala, Sweden 4 LERMA, Observatoire de Paris, PSL Research University, CNRS, Sorbonne Université, UPMC Université Paris 06, 75014 Paris, France 5 Niels Bohr Institute, University of Copenhagen, Øster Voldgade 5–7, 1350 Copenhagen K., Denmark 6 Institut de Radioastronomie Millimétrique, 38406 Saint-Martin d’Hères, France Received 7 June 2019 / Accepted 19 September 2019 ABSTRACT Methanol is a key species in astrochemistry because it is the most abundant organic molecule in the interstellar medium and is thought to be the mother molecule of many complex organic species. -
Methanol—Inhibitor Or Promoter of the Formation of Gas Hydrates from Deuterated Ice?
BOBEV AND TAIT: METHANOL—INHIBITOR OR PROMOTER? 1209 American Mineralogist, Volume 89, pages 1208–1214, 2004 Methanol—inhibitor or promoter of the formation of gas hydrates from deuterated ice? SVILEN BOBEV* AND KIMBERLY T. TAIT Manuel Lujan Jr. Neutron Scattering Center, LANSCE 12 MS H805, Los Alamos National Laboratory, Los Alamos, New Mexico 87545, U.S.A. ABSTRACT Kinetic studies are reported of the effect of methanol on the rate of formation of CO2- and CH4-hy- drates by means of in situ time-of-flight neutron powder diffraction. The experiments were carried out at temperatures ranging from 200 to 250 K and pressures up to 7 MPa. The samples were prepared from mixtures of ground, deuterated ice and deuterated methanol (up to 20 vol%), which were transformed in situ into CO2- or CH4-hydrates by pressurizing the systems with the corresponding gas. The observed rates of formation of hydrates are orders of magnitude higher than the rate of formation from pure deuterated ice under the same pressure and temperature conditions. Glycols and alcohols, methanol in particular, are long known as thermodynamic inhibitors of hydrate formation. Our study indicates that methanol can also act as a kinetic promoter for the formation of gas hydrates. Preliminary data suggest that the kinetics also depend strongly on concentration and the isotopic composition. INTRODUCTION particular, time-dependent neutron diffraction at a variety of tem- The crystal structures, thermodynamic models, and engineer- peratures and pressures has been effectively used to research the ing applications of CO2- and CH4-hydrates have been extensively kinetics of gas hydrate formation and dissociation (Henning et al. -
Hydrogen Isotope Exchanges Between Water and Methanol in Interstellar Ices a Faure, M Faure, P Theulé, E Quirico, B Schmitt
Hydrogen isotope exchanges between water and methanol in interstellar ices A Faure, M Faure, P Theulé, E Quirico, B Schmitt To cite this version: A Faure, M Faure, P Theulé, E Quirico, B Schmitt. Hydrogen isotope exchanges between water and methanol in interstellar ices. Astronomy and Astrophysics - A&A, EDP Sciences, 2015, 584, pp.A98. 10.1051/0004-6361/201526499. hal-01452286 HAL Id: hal-01452286 https://hal.archives-ouvertes.fr/hal-01452286 Submitted on 1 Feb 2017 HAL is a multi-disciplinary open access L’archive ouverte pluridisciplinaire HAL, est archive for the deposit and dissemination of sci- destinée au dépôt et à la diffusion de documents entific research documents, whether they are pub- scientifiques de niveau recherche, publiés ou non, lished or not. The documents may come from émanant des établissements d’enseignement et de teaching and research institutions in France or recherche français ou étrangers, des laboratoires abroad, or from public or private research centers. publics ou privés. A&A 584, A98 (2015) Astronomy DOI: 10.1051/0004-6361/201526499 & c ESO 2015 Astrophysics Hydrogen isotope exchanges between water and methanol in interstellar ices A. Faure1,2,M.Faure1,2, P. Theulé3, E. Quirico1,2, and B. Schmitt1,2 1 Univ. Grenoble Alpes, IPAG, 38000 Grenoble, France e-mail: [email protected] 2 CNRS, IPAG, 38000 Grenoble, France 3 Aix-Marseille Université, PIIM UMR-CNRS 7345, 13397 Marseille, France Received 8 May 2015 / Accepted 22 September 2015 ABSTRACT The deuterium fractionation of gas-phase molecules in hot cores is believed to reflect the composition of interstellar ices. -
H/D Exchange Processes in Flavonoids: Kinetics and Mechanistic Investigations
molecules Article H/D Exchange Processes in Flavonoids: Kinetics and Mechanistic Investigations Federico Bonaldo 1, Fulvio Mattivi 2 , Daniele Catorci 1,†, Panagiotis Arapitsas 3 and Graziano Guella 1,* 1 Bioorganic Chemistry Laboratory, Department of Physics, University of Trento, 38123 Trento, Italy; [email protected] (F.B.); [email protected] (D.C.) 2 Department of Cellular, Computational and Integrative Biology-CIBIO and C3A, University of Trento, 38123 Povo Trento, Italy; [email protected] 3 Department of Food Quality and Nutrition, Research and Innovation Centre, Fondazione Edmund Mach (FEM), 38098 San Michele all’Adige, Italy; [email protected] * Correspondence: [email protected] † D. Catorci passed away on 27 June 2020 while this manuscript was in the latest steps of preparation. Abstract: Several classes of flavonoids, such as anthocyanins, flavonols, flavanols, and flavones, undergo a slow H/D exchange on aromatic ring A, leading to full deuteration at positions C(6) and C(8). Within the flavanol class, H-C(6) and H-C(8) of catechin and epicatechin are slowly exchanged in D2O to the corresponding deuterated analogues. Even quercetin, a relevant flavonol representative, shows the same behaviour in a D2O/DMSOd6 1:1 solution. Detailed kinetic measurements of these H/D exchange processes are here reported by exploiting the time-dependent changes of their peak areas in the 1H-NMR spectra taken at different temperatures. A unifying reaction mechanism is also proposed based on our detailed kinetic observations, even taking into account pH and solvent effects. Molecular modelling and QM calculations were also carried out to shed more light on several molecular details of the proposed mechanism. -
Natural Products with Anticancer Activity from Moroccan Plant Thymelaea Lythroides and Its Endophyte Chaetomium Aureum
Natural Products with anticancer activity from Moroccan plant Thymelaea lythroides and its endophyte Chaetomium aureum Dissertation zur Erlangung des akademischen Grades Doktor der Naturwissenschaften (Dr. rer. nat.) und Doktor der Biowissenschaften und der Medizin (Dr.) vorgelegt der Bergischen Universität Wuppertal Fachbereich C – Mathematik und Naturwissenschaften und Mohammed V-Souissi Universität Rabat Fakultät – Medizin und Pharmazie von Fatima Zahra Kabbaj Wuppertal 2013 Aus der Arbeitsgruppe der Organischen Chemie der Bergischen Universität Wuppertal Diese Dissertation kann wie folgt zitiert werden: urn:nbn:de:hbz:468-20140404-113126-9 [http://nbn-resolving.de/urn/resolver.pl?urn=urn:nbn:de:hbz:468-20140404-113126-9] Gedruckt mit der Genehmigung der Mathematisch-Naturwissenschaftlichen Fachbereich C der Bergischen Universität Wuppertal und Medizinisch-Pharmazeutischen Fakultät der Mohammed V-Souissi Universität Rabat Referent: Prof. Dr. Hans-Josef Altenbach Koreferent: Prof. Dr. Moulay El Abbes Faouzi Tag der mündlichen Prüfung: 25.11.2013 Erklärung Hiermit erkläre ich ehrenwörtlich, dass ich die vorliegende Dissertation mit dem Titel „Natural Products with anticancer activity from Moroccan plant Thymelaea lythroides and its endophyte Chaetomium aureum“ selbst angefertigt habe. Außer den angegebenen Quellen und Hilfsmitteln wurden keine weiteren verwendet. Diese Dissertation wurde weder in gleicher noch in abgewandelter Form in einem anderen Prüfungsverfahren vorgelegt. Weiterhin erkläre ich, dass ich früher weder akademische Grade erworben habe, noch dies versucht habe. Wuppertal, den 21.10.2013 Fatima Zahra Kabbaj I dedicated this work to my husband and my parents Acknowledgement Acknowledgement First and foremost thanks to the Almighty God “ALLAH” who has granted me all these graces to fulfill this work and blessed me by His power, mercy and patience during my life. -
Towards the Topical Treatment of Psoriasis and Other Skin Diseases
1 Nanostructured supramolecular hydrogels: 2 towards the topical treatment of Psoriasis and 3 other skin diseases 4 David Limón,a,b* Kirian Talló Domínguez,a María Luisa Garduño,c Berenice Andrade,c Ana C. 5 Calpena b,d and Lluïsa Pérez-Garcíaa,b,e 6 aDepartament de Farmacologia, Toxicologia i Química Terapèutica, Universitat de Barcelona, Av. Joan 7 XXIII 27-31, 08028 Barcelona, Spain. 8 bInstitut de Nanociència i Nanotecnologia IN2UB, Universitat de Barcelona, 08028 Barcelona, Spain. 9 cCentro de Investigaciones Químicas, Universidad Autónoma del Estado de Morelos, Av. Universidad 10 1001, Cuernavaca, Morelos, México. 11 dDepartament de Farmàcia, Tecnologia Farmacèutica i Fisicoquímica, Universitat de Barcelona, Av. Joan 12 XXI, 27-31, 08028 Barcelona, Spain. 13 ePresent address: School of Pharmacy, The University of Nottingham, University Park, Nottingham 14 N672RD, United Kingdom. 15 ABSTRACT 16 Supramolecular hydrogels were synthesized using a bis-imidazolium based 17 amphiphile, and incorporating chemically diverse drugs, such as the cytostatics 18 gemcitabine hydrochloride and methotrexate sodium salt, the immunosuppressive 19 drug tacrolimus, as well as the corticoid drugs betamethasone 17-valerate and 20 triamcinolone acetonide, and their potential as drug delivery agents in the dermal 21 treatment of Psoriasis was evaluated. The rheological behavior of gels was studied, 22 showing in all cases suitable viscoelastic properties for topical drug delivery. Scanning 23 electron microscopy (SEM) shows that the drugs included have a great influence on 24 the gel morphology at the microscopic level, as the incorporation of gemcitabine 25 hydrochloride leads to slightly thicker fibers, the incorporation of tacrolimus induces 26 flocculation and spherical precipitates, and the incorporation of methotrexate forms 27 curled fibers. -
Agilent Vnmrj 4.2 Spectroscopy
Agilent VnmrJ 4.2 Spectroscopy User Guide Agilent Technologies Notices © Agilent Technologies, Inc. 2014 Warranty (June 1987) or DFAR 252.227-7015 (b)(2) (November 1995), as applicable in any No part of this manual may be reproduced The material contained in this docu- technical data. in any form or by any means (including ment is provided “as is,” and is sub- electronic storage and retrieval or transla- ject to being changed, without notice, tion into a foreign language) without prior Safety Notices agreement and written consent from in future editions. Further, to the max- Agilent Technologies, Inc. as governed by imum extent permitted by applicable United States and international copyright law, Agilent disclaims all warranties, CAUTION laws. either express or implied, with regard to this manual and any information A CAUTION notice denotes a haz- Manual Part Number contained herein, including but not ard. It calls attention to an operat- limited to the implied warranties of ing procedure, practice, or the like G7446-90573 merchantability and fitness for a par- that, if not correctly performed or ticular purpose. Agilent shall not be adhered to, could result in damage Edition liable for errors or for incidental or to the product or loss of important Revision B, June 2015 consequential damages in connection data. Do not proceed beyond a with the furnishing, use, or perfor- CAUTION notice until the indicated mance of this document or of any Revision log conditions are fully understood and information contained herein. Should met. Agilent and the user have a separate Revision Changes written agreement with warranty Rev A, May 2014 Initial revision for terms covering the material in this VnmrJ 4.2 document that conflict with these WARNING terms, the warranty terms in the sep- Rev B, June 2015 Minor corrections A WARNING notice denotes a arate agreement shall control. -
United States Patent (19) 11 Patent Number: 4,874,890 Kato Et Al
United States Patent (19) 11 Patent Number: 4,874,890 Kato et al. 45 Date of Patent: Oct. 17, 1989 54 PROCESS FOR THE PRODUCTION OF 56 References Cited DEUTERATED METHYL ACRYLATE OR PUBLICATIONS DEUTERATED METHYL METHACRYLATE Atkinson, Joseph G. et al. Chemical Abstracts (1972) 75 Inventors: Masaaki Kato; Tetsuya Uno; Masao #156,509e. Kobayashi; Naoto Osuga, all of Lockley, William J.S. Synth. Appl. Isot, Labeled Compa. Otake, Japan Proc. Int, Symp. (1982) Publ. 1983. 73) Assignee: Mitsubishi Rayon Co., Ltd., Tokyo, Lockley, William J. S. Chemical Abstracts vol. 98 Japan (1983) #215,266a. 21 Appl. No.: 154,485 Primary Examiner-Paul J. Killos 22 Filed: Feb. 8, 1988 Attorney, Agent, or Firm-Armstrong, Nikaido, Marmelstein, Kubovcik & Murray Related U.S. Application Data 57 ABSTRACT 63 Continuation of Ser. No. 807,710, Dec. 11, 1985, aban doned. There is provided the improved process for the produc tion of deutrated methyl acrylate or methacrylate by (30) Foreign Application Priority Data the direct substitution of deuterium for hydrogens in Dec. 21, 1984 JP Japan ................................ 59.270319 methyl acrylate or methacrylate in the presence of a platinum Group metal catalyst. 51 Int, Cl. ...... ... CO7C 69/52 52 U.S. Cl. .................................................... 560/205 58 Field of Search ......................................... 560/205 10 Claims, No Drawings 4,874,890 1. 2 at from room temperature to 300 C., but a temperature PROCESS FOR THE PRODUCTION OF from 50 to 150 C. is preferable from the standpoint o' DEUTERATED METHYL ACRYLATE OR reaction rate as well as the inhibition of side reaction: DEUTERATED METHYL METHACRYLATE and polymerization. -
Hydrogen Isotope Exchanges Between Water and Methanol In
Astronomy & Astrophysics manuscript no. 26499 c ESO 2018 October 16, 2018 Hydrogen isotope exchanges between water and methanol in interstellar ices A. Faure1,2, M. Faure1,2, P. Theul´e3, E. Quirico1,2, and B. Schmitt1,2 1 Univ. Grenoble Alpes, IPAG, F-38000 Grenoble, France 2 CNRS, IPAG, F-38000 Grenoble, France France e-mail: [email protected] 3 Aix-Marseille Universit´e, PIIM UMR-CNRS 7345, F-13397 Marseille, France Received / Accepted ABSTRACT The deuterium fractionation of gas-phase molecules in hot cores is believed to reflect the composition of interstellar ices. The deuter- ation of methanol is a major puzzle, however, because the isotopologue ratio [CH2DOH]/[CH3OD], which is predicted to be equal to 3 by standard grain chemistry models, is much larger ( 20) in low-mass hot corinos and significantly lower ( 1) in high-mass hot cores. This dichotomy in methanol deuteration between∼ low-mass and massive protostars is currently not understood.∼ In this study, we report a simplified rate equation model of the deuterium chemistry occurring in the icy mantles of interstellar grains. We apply this model to the chemistry of hot corinos and hot cores, with IRAS 16293-2422 and the Orion KL Compact Ridge as prototypes, respectively. The chemistry is based on a statistical initial deuteration at low temperature followed by a warm-up phase during which thermal hydrogen/deuterium (H/D) exchanges occur between water and methanol. The exchange kinetics is incorporated using lab- oratory data. The [CH2DOH]/[CH3OD] ratio is found to scale inversely with the D/H ratio of water, owing to the H/D exchange equilibrium between the hydroxyl (-OH) functional groups of methanol and water. -
Part 2. Mechanistic Aspects of the Reduction of S-Alkyl-Thionocarbonates in the Presence of Triethylborane and Air
Part 2. Mechanistic aspects of the reduction of S-alkyl-thionocarbonates in the presence of triethylborane and air Florent Allais1,2, Jean Boivin*1 and Van Tai Nguyen*1,3 Full Research Paper Open Access Address: Beilstein Journal of Organic Chemistry 2007, 3, No. 46. 1Institut de Chimie des Substances Naturelles, C.N.R.S., Avenue de doi:10.1186/1860-5397-3-46 la Terrasse, 91198 Gif-sur-Yvette, France, 2Institut National de la Recherche Agronomique, Centre de Versailles, Route de St-Cyr, Received: 26 September 2007 78026 Versailles, France and 3National Institute of Medicine Accepted: 12 December 2007 Materials, 3B, Quang Trung, Hanoi, Vietnam Published: 12 December 2007 Email: © 2007 Allais et al; licensee Beilstein-Institut Florent Allais - [email protected]; Jean Boivin* - License and terms: see end of document. [email protected]; Van Tai Nguyen* - [email protected] * Corresponding author Abstract Experiments conducted with deuterated compounds demonstrated that during the reduction of S-alkylxanthates with triethylborane, the hydrogen atom transferred has several competing origins. Hydrogen abstraction from water (or an alcohol) is the most favour- able route. Background In the first part of this series,[1] we showed that trialkylboranes very briefly that when Bu3SnH was omitted, the reduction still and especially commercial solutions of Et3B are efficient redu- occurred. The O-cyclododecyl S-methylxanthate derived from cing agents that permit the conversion, at room temperature, of cyclododecanol afforded cyclododecane in a remarkable 62% S-alkylxanthates, iodides and O-alkylxanthates into the corres- yield. No hypothesis about the origin of the hydrogen atom that ponding alkanes with good to excellent yields.