The Cold-Inducible RNA-Binding Motif Protein 3 (RBM3) Prevents Cell Death Through Various Mechanisms
Total Page:16
File Type:pdf, Size:1020Kb
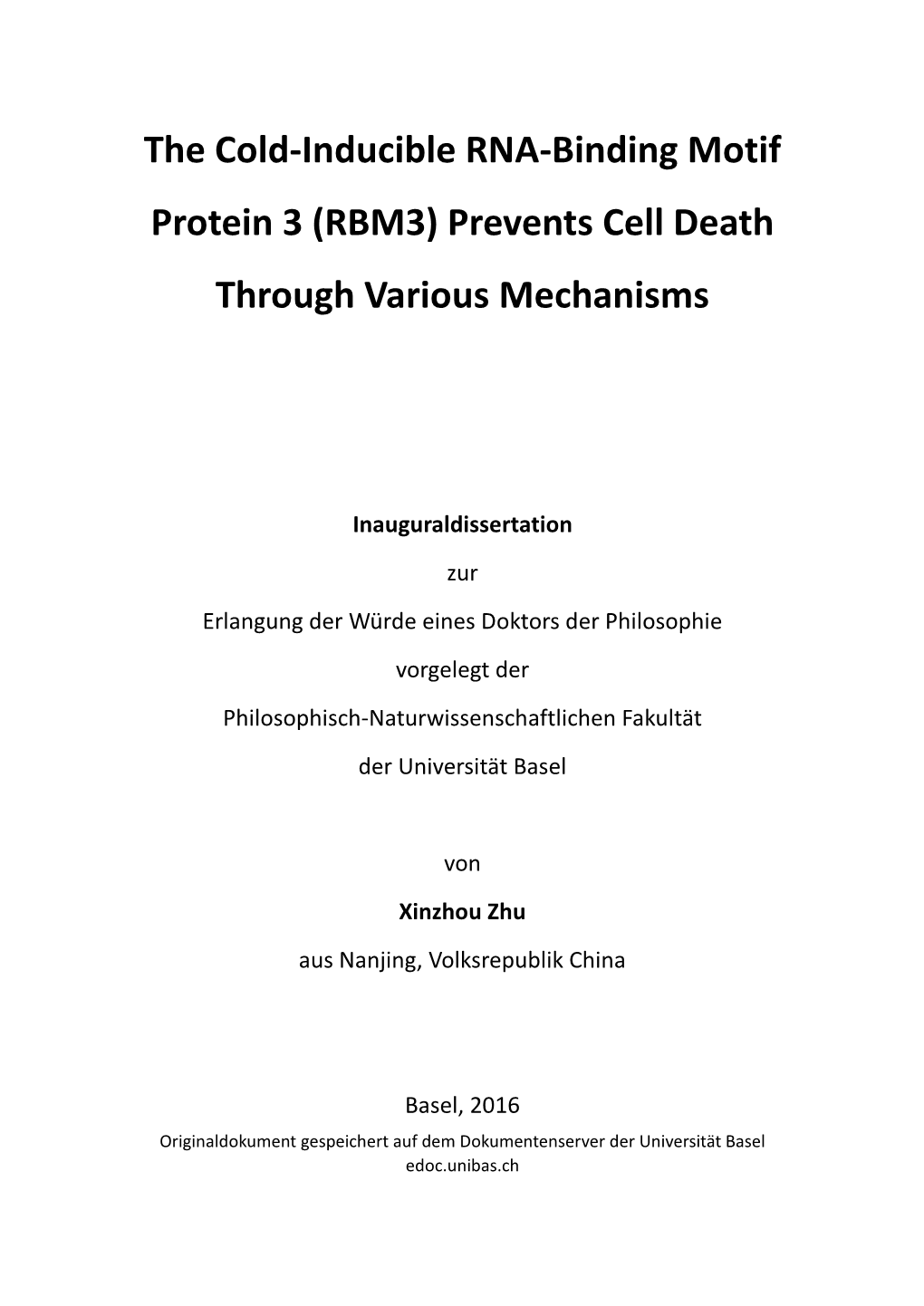
Load more
Recommended publications
-
Anti-RBM3 Antibody [HB9] (ARG23691)
Product datasheet [email protected] ARG23691 Package: 50 μg anti-RBM3 antibody [HB9] Store at: -20°C Summary Product Description Mouse Monoclonal antibody [HB9] recognizes RBM3 Tested Reactivity Hu Tested Application ICC/IF Specificity The antibody reacts against the recombinant human RBM3 full length under oxidative conditions without DTT or under reducing conditions with DTT. Cross reactivity of the antibody with the recombinant human Cold inducible Binding protein (CIRBP, full length 20.5 kDa) could not be detected. The apparent MW of hRBM3 in SDS-PAGE is ~17 kDa. Host Mouse Clonality Monoclonal Clone HB9 Isotype IgG1 Target Name RBM3 Antigen Species Human Immunogen Human RNA-binding protein 3. Conjugation Un-conjugated Alternate Names RNPL; IS1-RNPL; RNA-binding motif protein 3; RNA-binding protein 3 Application Instructions Application table Application Dilution ICC/IF 1:50 Application Note * The dilutions indicate recommended starting dilutions and the optimal dilutions or concentrations should be determined by the scientist. Calculated Mw 17 kDa Properties Form Liquid Purification Purification with Protein G. The IgG1 fraction was purified by affinity chromatography. Buffer PBS (pH 7.4) and 0.01% Sodium azide. Preservative 0.01% Sodium azide Storage instruction For continuous use, store undiluted antibody at 2-8°C for up to a week. For long-term storage, aliquot and store at -20°C or below. Storage in frost free freezers is not recommended. Avoid repeated freeze/thaw cycles. Suggest spin the vial prior to opening. The antibody solution should be gently mixed before use. www.arigobio.com 1/2 Note For laboratory research only, not for drug, diagnostic or other use. -
Identification of the Rna Binding Protein Rbm3 As a Novel Effector of Β-Catenin Signaling and Colon Cancer Stem Cells
IDENTIFICATION OF THE RNA BINDING PROTEIN RBM3 AS A NOVEL EFFECTOR OF β-CATENIN SIGNALING AND COLON CANCER STEM CELLS By Anand Venugopal Submitted to the graduate degree program in Molecular and Integrative Physiology and the Graduate Faculty of the University of Kansas in partial fulfillment of the requirements for the degree of Doctor of Philosophy. ________________________________ Chairperson Shrikant Anant, Ph.D. ________________________________ Roy Jensen, M.D. ________________________________ Andrew Godwin, Ph.D. ________________________________ Danny Welch, Ph.D. ________________________________ John Wood, Ph.D. Date Defended: ________________________________March 14, 2014 The Dissertation Committee for Anand Venugopal certifies that this is the approved version of the following dissertation: IDENTIFICATION OF THE RNA BINDING PROTEIN RBM3 AS A NOVEL EFFECTOR OF β-CATENIN SIGNALING AND COLON CANCER STEM CELLS ________________________________ Chairperson Shrikant Anant, Ph.D. Date approved: ________________________________March 14, 2014 ii Abstract The intestinal epithelium is one of the fastest renewing tissues within the adult. This renewal is primarily driven by the intestinal epithelial stem cell compartment and homeostasis of this compartment needs to be strictly maintained. Loss of regulation can lead to hyperplasia and subsequent malignant transformation. Moreover, cancers are also thought to contain a stem cell population which maintains long term tumor viability and recurrence following therapy. Therefore, a thorough understanding of the molecular machinery that governs stem cell homeostasis can further our understanding of colon cancer initiation and progression. The RNA binding protein RBM3 is upregulated in many solid tumors including colon, prostate and breast. It also serves as a proto-oncogene inducing the malignant transformation of normal cells when overexpressed. To further characterize the mechanism, we overexpressed RBM3 in DLD-1 and HCT 116 colon cancer cell lines. -
Human RBM3 ELISA Kit (ARG81349)
Product datasheet [email protected] ARG81349 Package: 96 wells Human RBM3 ELISA Kit Store at: 4°C Summary Product Description ARG81349 Human RBM3 ELISA Kit is an Enzyme Immunoassay kit for the quantification of Human RBM3 in serum and plasma. Tested Reactivity Hu Tested Application ELISA Specificity No significant cross reactivity to Cold inducible Binding protein (CIRBP), human. Target Name RBM3 Conjugation HRP Conjugation Note Substrate: TMB and read at 450 nm. Sensitivity 10 pg/ml Sample Type Serum and plasma. Standard Range 31.25 - 2000 pg/ml Sample Volume 50 µl Alternate Names RNPL; IS1-RNPL; RNA-binding motif protein 3; RNA-binding protein 3 Application Instructions Assay Time ~ 2.5 hours Properties Form 96 well Storage instruction Store the kit at 2-8°C. Keep microplate wells sealed in a dry bag with desiccants. Do not expose test reagents to heat, sun or strong light during storage and usage. Please refer to the product user manual for detail temperatures of the components. Note For laboratory research only, not for drug, diagnostic or other use. Bioinformation Gene Symbol RBM3 Gene Full Name RNA binding motif (RNP1, RRM) protein 3 Background This gene is a member of the glycine-rich RNA-binding protein family and encodes a protein with one RNA recognition motif (RRM) domain. Expression of this gene is induced by cold shock and low oxygen tension. A pseudogene exists on chromosome 1. Multiple alternatively spliced transcript variants that are predicted to encode different isoforms have been characterized although some of these variants fit nonsense-mediated decay (NMD) criteria. -
Human RBM3 ELISA Assay Kit
Human RBM3 ELISA Assay Kit BTRBM-001 For quantitative determination of human RNA-binding protein 3 96 Determinations 2°C – 8 °C For research use only Not for diagnostic use RBM3 ELISA Assay Kit 1 of 14 Catalog Number: BTRBM001 www.EagleBio.com Contents Short Review 3 Intended Use 4 Test principle 5 Safety warning and precautions 5 Storage 6 Components of the assay system 6 Sample preparation and storage 7 Critical parameters 7 Preparation of reagents 8 Assay protocol 8 Protocol summary 9 Scheme of the plate 10 Data processing 10 Additional information 11 Specificity 11 Sensitivity 11 Linearity 11 Recovery 11 Reproducibility 12 Troubleshooting 13 Related Products 14 BioTeZ Berlin-Buch GmbH Robert-Rössle-Str. 10, Haus 72 13125 Berlin Tel.: ++49 (0)30-9489 3322 Fax: ++49 (0)30-949 2008 e-mail: [email protected] http://www.biotez.de This manual is valid from May, 2016 RBM3 ELISA Assay Kit 2 of 14 Catalog Number: BTRBM001 www.EagleBio.com Short Review The RNA-binding protein 3 (RBM3) is a member of the glycine-rich RNA-binding protein family. The exact role of the cold shock protein RBM3 is not yet clear [2, 6]. The role of mRNA-binding proteins can be attributed to a regulation of mRNA splicing, stability, transport and ultimately the translation of mRNA into proteins. RBM3 consists of 157 amino acids with a predicted mass of 17 kD (1, 2) and is one of the few proteins that are upregulated at low temperature [3-5]. It is known that RBM3 acts especially in cellular stress (eg. -
Molecular Interactions Underpinning the Phenotype of Hibernation in Mammals Matthew T
© 2019. Published by The Company of Biologists Ltd | Journal of Experimental Biology (2019) 222, jeb160606. doi:10.1242/jeb.160606 REVIEW Molecular interactions underpinning the phenotype of hibernation in mammals Matthew T. Andrews* ABSTRACT most mammals. This Review covers recent advances in the Mammals maintain a constant warm body temperature, facilitating a molecular biology of hibernation, with a focus on molecular wide variety of metabolic reactions. Mammals that hibernate have the interactions underpinning the hibernation phenotype. Specific – ability to slow their metabolism, which in turn reduces their body topics include the torpor arousal cycle, the role of small temperature and leads to a state of hypothermic torpor. For this molecules, changes in gene expression, cold-inducible RNA- metabolic rate reduction to occur on a whole-body scale, molecular binding proteins, the somatosensory system and emerging interactions that change the physiology of cells, tissues and organs information on hibernating primates. This new information not are required, resulting in a major departure from normal mammalian only is beginning to explain how natural hibernators survive homeostasis. The aim of this Review is to cover recent advances in the physiological extremes that would be lethal to most mammals, but molecular biology of mammalian hibernation, including the role of also identifies molecular mechanisms that may prove useful to small molecules, seasonal changes in gene expression, cold- human medicine. inducible RNA-binding proteins, -
Oxygen-Regulated Expression of the RNA-Binding Proteins RBM3 and CIRP by a HIF-1-Independent Mechanism
Research Article 1785 Oxygen-regulated expression of the RNA-binding proteins RBM3 and CIRP by a HIF-1-independent mechanism Sven Wellmann1, Christoph Bührer2,*, Eva Moderegger1, Andrea Zelmer1, Renate Kirschner1, Petra Koehne2, Jun Fujita3 and Karl Seeger1 1Department of Pediatric Oncology/Hematology and the 2Department of Neonatology, Charité Campus Virchow-Klinikum, Medical University of Berlin, 13353 Berlin, Germany 3Department of Clinical Molecular Biology, Kyoto University, Kyoto 606-8507, Japan *Author for correspondence (e-mail: [email protected]) Accepted 1 December 2003 Journal of Cell Science 117, 1785-1794 Published by The Company of Biologists 2004 doi:10.1242/jcs.01026 Summary The transcriptional regulation of several dozen genes in target. In contrast, iron chelators induced VEGF but not response to low oxygen tension is mediated by hypoxia- RBM3 or CIRP. The RBM3 and CIRP mRNA increase after inducible factor 1 (HIF-1), a heterodimeric protein hypoxia was inhibited by actinomycin-D, and in vitro composed of two subunits, HIF-1α and HIF-1β. In the HIF- nuclear run-on assays demonstrated specific increases in 1α-deficient human leukemic cell line, Z-33, exposed to RBM3 and CIRP mRNA after hypoxia, which suggests that mild (8% O2) or severe (1% O2) hypoxia, we found regulation takes place at the level of gene transcription. significant upregulation of two related heterogenous Hypoxia-induced RBM3 or CIRP transcription was nuclear ribonucleoproteins, RNA-binding motif protein 3 inhibited by the respiratory chain inhibitors NaN3 and (RBM3) and cold inducible RNA-binding protein (CIRP), cyanide in a dose-dependent fashion. However, cells which are highly conserved cold stress proteins with RNA- depleted of mitochondria were still able to upregulate binding properties. -
RBM3: a Prognostic and Treatment Predictive Biomarker
RBM3: A Prognostic and Treatment Predictive Biomarker Introduction it has also been shown that RBM3 attenu- Cancer is continuously a major health ates stem cell-like properties in prostate problem throughout the world. In 2008, cancer cells6. there were an estimated 12.7 million new cancer cases world-wide, and 7.6 RBM3 monoclonal antibody million deaths that could be attributed to The monoclonal Anti-RBM3 antibody cancer1. Due to a world population with AMAb90655, used in all cases presented an increased life-expectancy, there is no here (available for purchase from Atlas reason to expect a decline in cancer inci- Antibodies, Stockholm, Sweden, atlasan- dence in the near future2. tibodies.com), has shown excellent spe- cificity in Western Blot analysis of human Cancer, though often denoted as a sin- cell lines, and is routinely used for stain- gular disease, is truly a multitude of dis- ing of formalin fixed paraffin embedded eases. This understanding has evolved tissue in IHC. A representative image of over the years, and though common immunohistochemical staining using the knowledge today, many patients are still RBM3 monoclonal antibody AMAb90655 not receiving optimal treatment for their can be seen in Figure 1. disease. For cancer patients to receive a more individualized treatment, there is RBM3 as a prognostic biomarker still a need for new and better ways to After identification of RBM3 as a poten- stratify patients. The classical, pathol- tial prognostic biomarker, RBM3 protein ogy based, prognostic factors such as expression has been analyzed using stage and grade of the tumor are insuf- AMAb90655 in many different patient co- ficient for a correct estimation of patient horts from various forms of cancer. -
Effect of Temperature Downshift on the Transcriptomic Responses of Chinese Hamster Ovary Cells Using Recombinant Human Tissue Plasminogen Activator Production Culture
RESEARCH ARTICLE Effect of Temperature Downshift on the Transcriptomic Responses of Chinese Hamster Ovary Cells Using Recombinant Human Tissue Plasminogen Activator Production Culture Andrea Bedoya-López1, Karel Estrada2, Alejandro Sanchez-Flores2, Octavio T. Ramírez3, Claudia Altamirano4, Lorenzo Segovia5, Juan Miranda-Ríos1, Mauricio A. Trujillo-Roldán1, Norma A. Valdez-Cruz1* 1 Departamento de Biología Molecular y Biotecnología, Instituto de Investigaciones Biomédicas, Universidad Nacional Autónoma de México, Ciudad de México, México, 2 Unidad Universitaria de Apoyo Bioinformático, Instituto de Biotecnología, Universidad Nacional Autónoma de México, Cuernavaca, Mor. México, 3 Departamento de Medicina Molecular y Bioprocesos, Instituto de Biotecnología, Universidad Nacional Autónoma de México, Cuernavaca, Mor. México, 4 Escuela de Ingeniería Bioquímica, Pontificia OPEN ACCESS Universidad Católica de Valparaíso, Valparaíso, Chile, 5 Departamento de Ingeniería Celular y Biocatálisis. Instituto de Biotecnología, Universidad Nacional Autónoma de México, Cuernavaca, Mor. México Citation: Bedoya-López A, Estrada K, Sanchez- Flores A, Ramírez OT, Altamirano C, Segovia L, et al. * [email protected] (2016) Effect of Temperature Downshift on the Transcriptomic Responses of Chinese Hamster Ovary Cells Using Recombinant Human Tissue Plasminogen Activator Production Culture. PLoS Abstract ONE 11(3): e0151529. doi:10.1371/journal. pone.0151529 Recombinant proteins are widely used as biopharmaceuticals, but their production by mam- Editor: Jonathan -
Impact of RNA‑Binding Motif 3 Expression on the Whole Transcriptome of Prostate Cancer Cells: an RNA Sequencing Study
ONCOLOGY REPORTS 40: 2307-2315, 2018 Impact of RNA‑binding motif 3 expression on the whole transcriptome of prostate cancer cells: An RNA sequencing study QINGZHUO DONG1, CHENGCHENG LV1, GEJUN ZHANG1,2, ZI YU1,2, CHUIZE KONG2, CHENG FU1 and YU ZENG1 1Department of Urology, Cancer Hospital of China Medical University, Liaoning Cancer Hospital and Institute, Shenyang, Liaoning 110042; 2Department of Urology, The First Hospital of China Medical University, Shenyang, Liaoning 110001, P.R. China Received March 3, 2018; Accepted July 25, 2018 DOI: 10.3892/or.2018.6618 Abstract. RNA-binding motif 3 (RBM3) is a cold-shock Introduction protein that has been previously shown to attenuate cancer stem cell-like features in prostate cancer (PCa) cells. However, There were 4,292,000 new cases of cancer and 2,814,000 cases the mechanism underlying RBM3 regulation in PCa cells is of cancer-associated mortality reported in 2015 in China (1). largely unknown. The present study investigated the impact Among all types of cancer, prostate cancer (PCa) was diag- of RBM3 expression on the whole transcriptome of PCa nosed in ~603,000 men, and over 26,000 men succumbed to cells using high-throughput RNA sequencing (RNA-seq). this disease (1). Although the incidence and mortality rates of Differentially expressed genes (DEGs) that were identified PCa remain lower in China than in Western countries, they through RNA-seq were applied to Gene Ontology (GO), are increasing rapidly. Thus, PCa has become one of the major pathway analysis, pathway-action networks and protein-protein healthy issues for men in China. -
Oncology Biomarkers - Assessing Cancer Development Oncology Biomarkers
Oncology Biomarkers - Assessing Cancer Development Oncology Biomarkers Need for Novel Cancer Biomarkers With a limited repertoire of protein biomar- Biomarkers for Licensing The microscopic evaluation of stained tis- ers available today there is a clear and un- Several proprietary oncology biomarkers sue sections from a tumor remains the met clinical need to identify novel sets of are available for licencing from Atlas Anti- gold standard for cancer diagnosis. How- biomarkers. The aim is to provide a more bodies, both Triple A Polyclonals and Pre- ever, in order to optimize patient treatment accurate diagnosis and a better assess- cisA Monoclonals. A selection of available and provide guidance for therapeutic in- ment of patient prognosis, ultimately lead- antibodies is presented below. tervention of the underlying disease there ing to a more individualized treatment. is often a need for additional tumor stratifi- Additional information on licensing of spe- cation methods. Atlas Antibodies recognize this need for cific biomarkers and information about At- new biomarkers. Together with our re- las Antibodies’ customized solutions and The analysis of protein expression in cells search partners in the Human Protein validation capabilities is available on re- from a tumor tissue often provides impor- Atlas project we are in a unique position quest by sending an email to: tant additional information to the patholo- to perform antibody-based biomarker dis- [email protected] or visit our gist. Immunohistochemistry (IHC) using covery. -
RBMX Family Proteins Connect the Fields of Nuclear RNA Processing
International Journal of Biochemistry and Cell Biology 108 (2019) 1–6 Contents lists available at ScienceDirect International Journal of Biochemistry and Cell Biology journal homepage: www.elsevier.com/locate/biocel RBMX family proteins connect the fields of nuclear RNA processing, disease and sex chromosome biology T ⁎ David J. Elliott , Caroline Dalgliesh, Gerald Hysenaj, Ingrid Ehrmann Institute of Genetic Medicine, Newcastle University, Newcastle-upon-Tyne, NE1 3BZ, UK ARTICLE INFO ABSTRACT Keywords: RBMX is a ubiquitously expressed nuclear RNA binding protein that is encoded by a gene on the X chromosome. RNA splicing RBMX belongs to a small protein family with additional members encoded by paralogs on the mammalian Y Gene expression chromosome and other chromosomes. These RNA binding proteins are important for normal development, and Brain development also implicated in cancer and viral infection. At the molecular level RBMX family proteins contribute to splicing Testis control, transcription and genome integrity. Establishing what endogenous genes and pathways are controlled by Cancer RBMX and its paralogs will have important implications for understanding chromosome biology, DNA repair and mammalian development. Here we review what is known about this family of RNA binding proteins, and identify important current questions about their functions. 1. Introduction et al., 1993). While RBMX is expressed ubiquitously through the body, RBMY genes are specifically expressed in germ cells (cells that even- RBMX (an acronym of RNA Binding Motif protein, X-linked) was tually develop into sperm). Multiple RBMY genes are present on the originally identified as one of a functionally diverse group of nuclear long arm of the human Y chromosome, each encoding 496 amino acid proteins that bind to polyadenylated RNA. -
(RBM3) Regulates Type 2 Innate Lymphoid Cell (ILC2) Cytokine Production
UNIVERSITY OF CALIFORNIA, SAN DIEGO RNA-Binding Motif 3 (RBM3) regulates Type 2 Innate Lymphoid cell (ILC2) Cytokine Production A Thesis Submitted in Partial Satisfaction of the Requirements for the Degree Master of Science in Biology by Jana Hicham Badrani Committee in charge: Taylor A. Doherty, Chair Li-Fan Lu, Co-Chair James Cooke 2017 Copyright Jana Hicham Badrani, 2017 All rights reserved. The Thesis of Jana Hicham Badrani is approved, and it is acceptable in quality and form for publication on microfilm and electronically: Co-Chair Chair University of California, San Diego 2017 iii DEDICATION This work is dedicated to my family: my parents, for their unwavering faith and drive, and my siblings, for their never ending curiosity and constant criticism. My parents have constantly stood by me and have always supported my dreams and desires. They have provided me with an appreciation of hard work and the drive to progress further than I ever could alone. They are, and always will be, my inspiration, my motivation, and my best friends. My younger siblings are constant reminders of life’s simple pleasures. They have shown me how far an active imagination can take you and have taught me all I know about patience. iv TABLE OF CONTENTS Signature Page…………………………………………………...……………………….iii Dedication………………………………………………………………………………...iv Table of Contents………………………………………………………………………….v List of Figures………………………………………………………………………….…vi Acknowledgements………………………………………………………………………vii Abstract of the Thesis…………………………………………………………………...viii Introduction………………………………………………………………………………..1