Regulation of BCR-ABL Expression Via Its 3' Untranslated Region
Total Page:16
File Type:pdf, Size:1020Kb
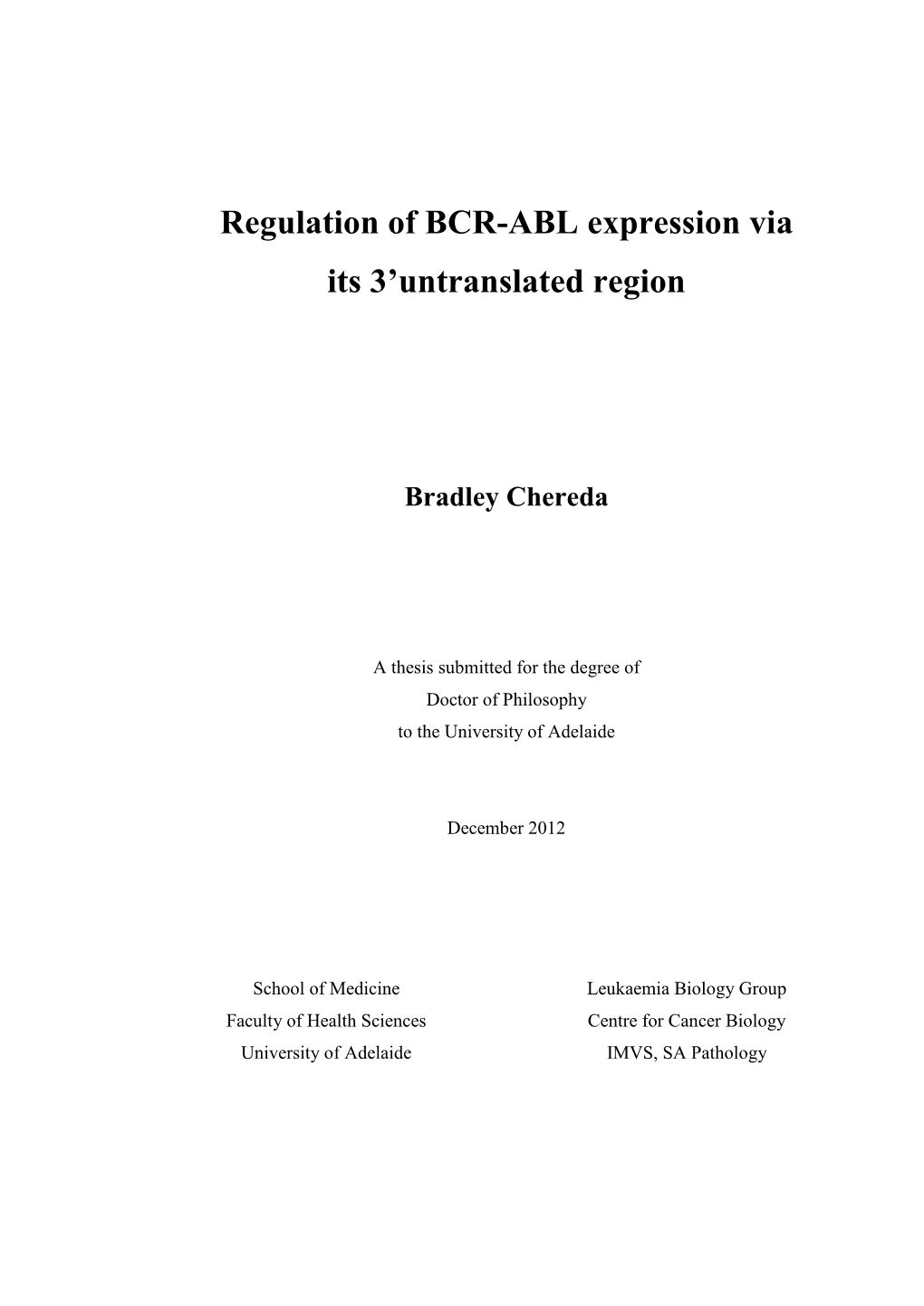
Load more
Recommended publications
-
SRC Antibody - N-Terminal Region (ARP32476 P050) Data Sheet
SRC antibody - N-terminal region (ARP32476_P050) Data Sheet Product Number ARP32476_P050 Product Name SRC antibody - N-terminal region (ARP32476_P050) Size 50ug Gene Symbol SRC Alias Symbols ASV; SRC1; c-SRC; p60-Src Nucleotide Accession# NM_005417 Protein Size (# AA) 536 amino acids Molecular Weight 60kDa Product Format Lyophilized powder NCBI Gene Id 6714 Host Rabbit Clonality Polyclonal Official Gene Full Name V-src sarcoma (Schmidt-Ruppin A-2) viral oncogene homolog (avian) Gene Family SH2D This is a rabbit polyclonal antibody against SRC. It was validated on Western Blot by Aviva Systems Biology. At Aviva Systems Biology we manufacture rabbit polyclonal antibodies on a large scale (200-1000 Description products/month) of high throughput manner. Our antibodies are peptide based and protein family oriented. We usually provide antibodies covering each member of a whole protein family of your interest. We also use our best efforts to provide you antibodies recognize various epitopes of a target protein. For availability of antibody needed for your experiment, please inquire (). Peptide Sequence Synthetic peptide located within the following region: QTPSKPASADGHRGPSAAFAPAAAEPKLFGGFNSSDTVTSPQRAGPLAGG This gene is highly similar to the v-src gene of Rous sarcoma virus. This proto-oncogene may play a role in the Description of Target regulation of embryonic development and cell growth. SRC protein is a tyrosine-protein kinase whose activity can be inhibited by phosphorylation by c-SRC kinase. Mutations in this gene could be involved in the -
A Computational Approach for Defining a Signature of Β-Cell Golgi Stress in Diabetes Mellitus
Page 1 of 781 Diabetes A Computational Approach for Defining a Signature of β-Cell Golgi Stress in Diabetes Mellitus Robert N. Bone1,6,7, Olufunmilola Oyebamiji2, Sayali Talware2, Sharmila Selvaraj2, Preethi Krishnan3,6, Farooq Syed1,6,7, Huanmei Wu2, Carmella Evans-Molina 1,3,4,5,6,7,8* Departments of 1Pediatrics, 3Medicine, 4Anatomy, Cell Biology & Physiology, 5Biochemistry & Molecular Biology, the 6Center for Diabetes & Metabolic Diseases, and the 7Herman B. Wells Center for Pediatric Research, Indiana University School of Medicine, Indianapolis, IN 46202; 2Department of BioHealth Informatics, Indiana University-Purdue University Indianapolis, Indianapolis, IN, 46202; 8Roudebush VA Medical Center, Indianapolis, IN 46202. *Corresponding Author(s): Carmella Evans-Molina, MD, PhD ([email protected]) Indiana University School of Medicine, 635 Barnhill Drive, MS 2031A, Indianapolis, IN 46202, Telephone: (317) 274-4145, Fax (317) 274-4107 Running Title: Golgi Stress Response in Diabetes Word Count: 4358 Number of Figures: 6 Keywords: Golgi apparatus stress, Islets, β cell, Type 1 diabetes, Type 2 diabetes 1 Diabetes Publish Ahead of Print, published online August 20, 2020 Diabetes Page 2 of 781 ABSTRACT The Golgi apparatus (GA) is an important site of insulin processing and granule maturation, but whether GA organelle dysfunction and GA stress are present in the diabetic β-cell has not been tested. We utilized an informatics-based approach to develop a transcriptional signature of β-cell GA stress using existing RNA sequencing and microarray datasets generated using human islets from donors with diabetes and islets where type 1(T1D) and type 2 diabetes (T2D) had been modeled ex vivo. To narrow our results to GA-specific genes, we applied a filter set of 1,030 genes accepted as GA associated. -
Systematic Description of the Expression and Prognostic Value of M6A Regulators in Human Ovarian Cancer
Systematic Description of the Expression and Prognostic Value of M6A Regulators in Human Ovarian Cancer Yuwei Chen Fujian Medical University Fujian Cancer Hospital Yang Sun ( [email protected] ) Fujian Cancer Hospital https://orcid.org/0000-0003-4224-8832 Xinbei Chen Fujian Medical University Fujian Cancer Hospital Siming Li Fujian Medical University Fujian Cancer Hospital Hongmei Xia Fujian Cancer Hospital Research Keywords: m6A regulators, m6A, ovarian cancer, Prognostic values, bioinformatics analysis. Posted Date: December 22nd, 2020 DOI: https://doi.org/10.21203/rs.3.rs-131682/v1 License: This work is licensed under a Creative Commons Attribution 4.0 International License. Read Full License Systematic description of the expression and prognostic value of m6A regulators in human ovarian cancer 1 Yuwei Chen1, Yang Sun1*, Xinbei Chen1, Siming Li1, Hongmei Xia1 2 * Correspondence: 3 Yang Sun 4 [email protected] 5 Total words:5253 6 Total Figures and Tables: 11 Figures and 3 Tables 7 Keywords: m6A regulators, m6A, ovarian cancer, Prognostic values, 8 bioinformatics analysis. 9 Abstract 10 Background: N6-methyladenine (m6A) methylation, known as a kind of RNA 11 methylation regulator, has become a hotspot for research in the life sciences in recent 12 years. The existing studies revealed that m6A regulators helped to regulate the 13 progression of several malignant tumors. However, the expression mode and 14 prognostic value of m6A regulators in ovarian cancer have not been fully elucidated. 15 Methods: ONCOMINE, GEPIA, Human protein atlas, Kaplan-meier, UALCAN, 16 cBioPortal, GeneMANIA, DAVID, Sring, and Metascape were utilized in this study. 17 Results: In this study, through a comprehensive use of multiple database systems, 18 m6A regulators-induced mRNA and protein expression levels and their prognostic 19 value in the epithelial ovarian cancer of various histological types were analyzed. -
CRISPR-Cas9–Based Treatment of Myocilin-Associated Glaucoma
CRISPR-Cas9–based treatment of myocilin- associated glaucoma Ankur Jaina, Gulab Zodeb,1, Ramesh B. Kasettib, Fei A. Ranc, Winston Yanc, Tasneem P. Sharmad, Kevin Buggea, Charles C. Searbya, John H. Fingertd, Feng Zhangc, Abbot F. Clarkb, and Val C. Sheffielda,d,1 aDepartment of Pediatrics, Carver College of Medicine, University of Iowa, Iowa City, IA 52242; bNorth Texas Eye Research Institute, University of North Texas Health Science Center, Fort Worth, TX 76107; cMcGovern Institute for Brain Research, Massachusetts Institute of Technology, Cambridge, MA 02142; and dStephen A. Wynn Institute for Vision Research, Department of Ophthalmology, Carver College of Medicine, University of Iowa, Iowa City, IA 52242 Edited by Donald J. Zack, Johns Hopkins University, Baltimore, MD, and accepted by Editorial Board Member Jeremy Nathans August 25, 2017 (received for review April 22, 2017) Primary open-angle glaucoma (POAG) is a leading cause of protein itself (transcription or translational inhibition). While irreversible vision loss worldwide, with elevated intraocular pres- siRNA and shRNA provide potentially viable treatment op- sure (IOP) a major risk factor. Myocilin (MYOC) dominant gain-of- tions (31), we elected to directly target the MYOC gene using function mutations have been reported in ∼4% of POAG cases. gene editing with clustered regularly interspaced short palindromic MYOC mutations result in protein misfolding, leading to endoplas- repeats (CRISPR)-Cas9 technology to treat myocilin-associated mic reticulum (ER) stress in the trabecular meshwork (TM), the tis- glaucoma. sue that regulates IOP. We use CRISPR-Cas9–mediated genome Originally part of the prokaryotic adaptive immune system, the editing in cultured human TM cells and in a MYOC mouse model CRISPR-Cas9 system has been adapted as a genome-editing tool, of POAG to knock down expression of mutant MYOC, resulting in in which the Cas9 endonuclease is directed by a guide RNA relief of ER stress. -
Supp Material.Pdf
Supplementary Information Estrogen-mediated Epigenetic Repression of Large Chromosomal Regions through DNA Looping Pei-Yin Hsu, Hang-Kai Hsu, Gregory A. C. Singer, Pearlly S. Yan, Benjamin A. T. Rodriguez, Joseph C. Liu, Yu-I Weng, Daniel E. Deatherage, Zhong Chen, Julia S. Pereira, Ricardo Lopez, Jose Russo, Qianben Wang, Coral A. Lamartiniere, Kenneth P. Nephew, and Tim H.-M. Huang S1 Method Immunofluorescence staining Approximately 2,000 mammosphere-derived epithelial cells (MDECs) cells seeded collagen I-coated coverslips were fixed with methanol/acetone for 10 min. After blocking with 2.5% bovine serum albumin (Sigma) for 1 hr, these cells were incubated with anti-ESR1 antibody (Santa Cruz) overnight at 4˚C. The corresponding secondary FITC-conjugated antibody was applied followed by DAPI staining (Molecular Probes) for the nuclei. Photographs were captured by Zeiss fluorescence microscopy (Zeiss). The percentages of ESR1 subcellular localization were calculated in ten different optical fields (~10 cells per field) by two independent researchers. References Carroll, J.S., Meyer, C.A., Song, J., Li, W., Geistlinger, T.R., Eeckhoute, J., Brodsky, A.S., Keeton, E.K., Fertuck, K.C., Hall, G.F., et al. 2006. Genome-wide analysis of estrogen receptor binding sites. Nat. Genet. 38: 1289-1297. Neve, R.M., Chin, K., Fridlyand, J., Yeh, J., Baehner, F.L., Fevr, T., Clark, L., Bayani, N., Coppe, J.P., Tong, F., et al. 2006. A collection of breast cancer cell lines for the study of functionally distinct cancer subtypes. Cancer Cell 10: 515-527. S2 Hsu et al. Supplementary Information A Figure S1. Integrative mapping of large genomic regions subjected to ERα-mediated epigenetic repression. -
Extensive Characterization of IFN-Induced Gtpases Mgbp1 to Mgbp10 Involved in Host Defense
Extensive Characterization of IFN-Induced GTPases mGBP1 to mGBP10 Involved in Host Defense This information is current as Daniel Degrandi, Carolin Konermann, Cornelia of October 1, 2021. Beuter-Gunia, Alexandra Kresse, Jan Würthner, Stefanie Kurig, Sandra Beer and Klaus Pfeffer J Immunol 2007; 179:7729-7740; ; doi: 10.4049/jimmunol.179.11.7729 http://www.jimmunol.org/content/179/11/7729 Downloaded from Supplementary http://www.jimmunol.org/content/suppl/2008/03/10/179.11.7729.DC1 Material http://www.jimmunol.org/ References This article cites 56 articles, 23 of which you can access for free at: http://www.jimmunol.org/content/179/11/7729.full#ref-list-1 Why The JI? Submit online. • Rapid Reviews! 30 days* from submission to initial decision • No Triage! Every submission reviewed by practicing scientists by guest on October 1, 2021 • Fast Publication! 4 weeks from acceptance to publication *average Subscription Information about subscribing to The Journal of Immunology is online at: http://jimmunol.org/subscription Permissions Submit copyright permission requests at: http://www.aai.org/About/Publications/JI/copyright.html Email Alerts Receive free email-alerts when new articles cite this article. Sign up at: http://jimmunol.org/alerts The Journal of Immunology is published twice each month by The American Association of Immunologists, Inc., 1451 Rockville Pike, Suite 650, Rockville, MD 20852 Copyright © 2007 by The American Association of Immunologists All rights reserved. Print ISSN: 0022-1767 Online ISSN: 1550-6606. The Journal of Immunology Extensive Characterization of IFN-Induced GTPases mGBP1 to mGBP10 Involved in Host Defense1 Daniel Degrandi,2 Carolin Konermann,2 Cornelia Beuter-Gunia,2 Alexandra Kresse, Jan Wu¨rthner, Stefanie Kurig, Sandra Beer,3 and Klaus Pfeffer3 IFN-␥ orchestrates a potent antimicrobial host response. -
WO 2019/079361 Al 25 April 2019 (25.04.2019) W 1P O PCT
(12) INTERNATIONAL APPLICATION PUBLISHED UNDER THE PATENT COOPERATION TREATY (PCT) (19) World Intellectual Property Organization I International Bureau (10) International Publication Number (43) International Publication Date WO 2019/079361 Al 25 April 2019 (25.04.2019) W 1P O PCT (51) International Patent Classification: CA, CH, CL, CN, CO, CR, CU, CZ, DE, DJ, DK, DM, DO, C12Q 1/68 (2018.01) A61P 31/18 (2006.01) DZ, EC, EE, EG, ES, FI, GB, GD, GE, GH, GM, GT, HN, C12Q 1/70 (2006.01) HR, HU, ID, IL, IN, IR, IS, JO, JP, KE, KG, KH, KN, KP, KR, KW, KZ, LA, LC, LK, LR, LS, LU, LY, MA, MD, ME, (21) International Application Number: MG, MK, MN, MW, MX, MY, MZ, NA, NG, NI, NO, NZ, PCT/US2018/056167 OM, PA, PE, PG, PH, PL, PT, QA, RO, RS, RU, RW, SA, (22) International Filing Date: SC, SD, SE, SG, SK, SL, SM, ST, SV, SY, TH, TJ, TM, TN, 16 October 2018 (16. 10.2018) TR, TT, TZ, UA, UG, US, UZ, VC, VN, ZA, ZM, ZW. (25) Filing Language: English (84) Designated States (unless otherwise indicated, for every kind of regional protection available): ARIPO (BW, GH, (26) Publication Language: English GM, KE, LR, LS, MW, MZ, NA, RW, SD, SL, ST, SZ, TZ, (30) Priority Data: UG, ZM, ZW), Eurasian (AM, AZ, BY, KG, KZ, RU, TJ, 62/573,025 16 October 2017 (16. 10.2017) US TM), European (AL, AT, BE, BG, CH, CY, CZ, DE, DK, EE, ES, FI, FR, GB, GR, HR, HU, ΓΕ , IS, IT, LT, LU, LV, (71) Applicant: MASSACHUSETTS INSTITUTE OF MC, MK, MT, NL, NO, PL, PT, RO, RS, SE, SI, SK, SM, TECHNOLOGY [US/US]; 77 Massachusetts Avenue, TR), OAPI (BF, BJ, CF, CG, CI, CM, GA, GN, GQ, GW, Cambridge, Massachusetts 02139 (US). -
A Novel Hypoxic Long Noncoding RNA KB-1980E6.3 Maintains Breast Cancer Stem Cell Stemness Via Interacting with IGF2BP1 to Facilitate C-Myc Mrna Stability
Oncogene (2021) 40:1609–1627 https://doi.org/10.1038/s41388-020-01638-9 ARTICLE A novel hypoxic long noncoding RNA KB-1980E6.3 maintains breast cancer stem cell stemness via interacting with IGF2BP1 to facilitate c-Myc mRNA stability 1 2 3 4 1 1 1 1 1 Pengpeng Zhu ● Fang He ● Yixuan Hou ● Gang Tu ● Qiao Li ● Ting Jin ● Huan Zeng ● Yilu Qin ● Xueying Wan ● 1 1 5 1 Yina Qiao ● Yuxiang Qiu ● Yong Teng ● Manran Liu Received: 15 June 2020 / Revised: 13 November 2020 / Accepted: 18 December 2020 / Published online: 19 January 2021 © The Author(s), under exclusive licence to Springer Nature Limited 2021. This article is published with open access Abstract The hostile hypoxic microenvironment takes primary responsibility for the rapid expansion of breast cancer tumors. However, the underlying mechanism is not fully understood. Here, using RNA sequencing (RNA-seq) analysis, we identified a hypoxia-induced long noncoding RNA (lncRNA) KB-1980E6.3, which is aberrantly upregulated in clinical breast cancer tissues and closely correlated with poor prognosis of breast cancer patients. The enhanced lncRNA KB- 1980E6.3 facilitates breast cancer stem cells (BCSCs) self-renewal and tumorigenesis under hypoxic microenvironment both 1234567890();,: 1234567890();,: in vitro and in vivo. Mechanistically, lncRNA KB-1980E6.3 recruited insulin-like growth factor 2 mRNA-binding protein 1 (IGF2BP1) to form a lncRNA KB-1980E6.3/IGF2BP1/c-Myc signaling axis that retained the stability of c-Myc mRNA through increasing binding of IGF2BP1 with m6A-modified c-Myc coding region instability determinant (CRD) mRNA. In conclusion, we confirm that lncRNA KB-1980E6.3 maintains the stemness of BCSCs through lncRNA KB-1980E6.3/ IGF2BP1/c-Myc axis and suggest that disrupting this axis might provide a new therapeutic target for refractory hypoxic tumors. -