A Dedicated Diribonucleotidase Resolves a Key Bottleneck for The
Total Page:16
File Type:pdf, Size:1020Kb
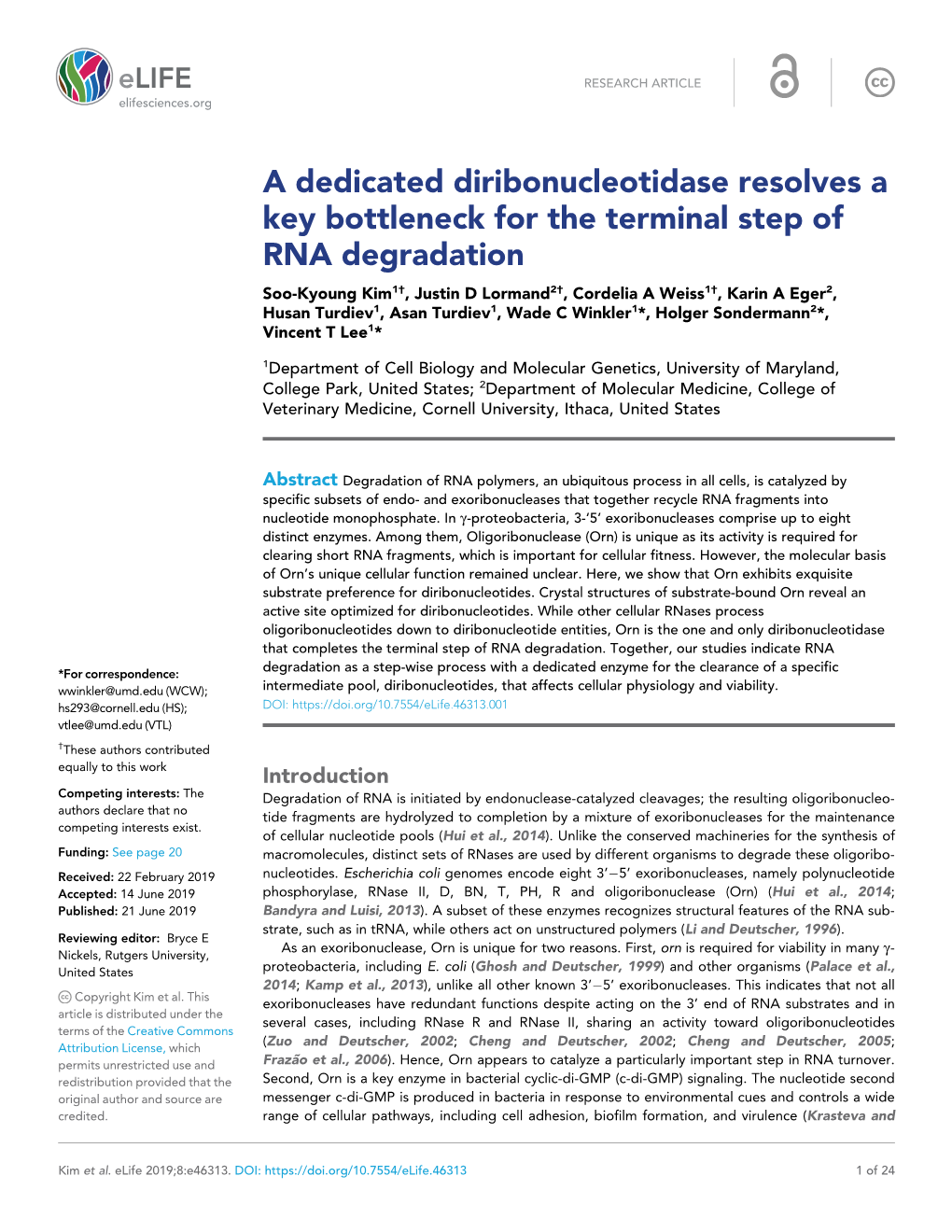
Load more
Recommended publications
-
Atypical 11Q Deletions Identified by Array CGH May Be Missed by FISH
Letters to the Editor 1011 1 2 2 2 1 AC Ng , SK Kumar , SJ Russell , SV Rajkumar and MT Drake 2 Axelrod L. Glucocorticoid therapy. Medicine (Baltimore) 1976; 55: 1 Division of Endocrinology, Department of Medicine, 39–65. Mayo Clinic, Rochester, MN, USA and 3 Krasner AS. Glucocorticoid-induced adrenal insufficiency. JAMA 2Division of Hematology, Department of Medicine, 1999; 282: 671–676. Mayo Clinic, Rochester, MN, USA 4 Schlaghecke R, Kornely E, Santen RT, Ridderskamp P. The effect of E-mail: [email protected] long-term glucocorticoid therapy on pituitary-adrenal responses to exogenous corticotropin-releasing hormone. N Engl J Med 1992; 326: 226–230. References 5 Grinspoon SK, Biller BM. Clinical review 62: laboratory assessment of adrenal insufficiency. J Clin Endocrinol Metab 1994; 79: 1 Rajkumar SV, Jacobus S, Callander N, Fonseca R, Vesole D, Williams 923–931. M et al. A randomized trial of lenalidomide plus high-dose 6 Nieman LK. Dynamic evaluation of adrenal hypofunction. dexamethasone (RD) versus lenalidomide plus low-dose dexametha- J Endocrinol Invest 2003; 26 (7 Suppl): 74–82. sone (Rd) in newly diagnosed multiple myeloma (E4A03): a trial 7 Hagg E, Asplund K, Lithner F. Value of basal plasma cortisol assays coordinated by the Eastern Cooperative Oncology Group. Blood in the assessment of pituitary-adrenal insufficiency. Clin Endocrinol (ASH Annual Meeting Abstracts) 2006; 108:799. (Oxf) 1987; 26: 221–226. Atypical 11q deletions identified by array CGH may be missed by FISH panels for prognostic markers in chronic lymphocytic leukemia Leukemia (2009) 23, 1011–1017; doi:10.1038/leu.2008.393; results were because of small clonal cell populations that published online 22 January 2009 comprised o25–30% of the total sample. -
PLZF Targets Developmental Enhancers for Activation During Osteogenic Differentiation of Human Mesenchymal Stem Cells
RESEARCH ARTICLE PLZF targets developmental enhancers for activation during osteogenic differentiation of human mesenchymal stem cells Shuchi Agrawal Singh1,2,3*, Mads Lerdrup1,3, Ana-Luisa R Gomes1,3, Harmen JG van de Werken4,5,6, Jens Vilstrup Johansen1,3,7, Robin Andersson1,3,7, Albin Sandelin1,3,7, Kristian Helin8,9,10, Klaus Hansen1,3* 1Biotech Research and Innovation Centre (BRIC), Faculty of Health and Medical Sciences, University of Copenhagen, Copenhagen, Denmark; 2Department of Hematology, Cambridge Institute for Medical Research and Welcome Trust/MRC Stem Cell Institute, University of Cambridge, Cambridge, United Kingdom; 3Centre for Epigenetics, Faculty of Health and Medical Sciences, University of Copenhagen, Copenhagen, Denmark; 4Department of Cell Biology, University Medical Center, Rotterdam, Netherlands; 5Cancer Computational Biology Center, University Medical Center, Rotterdam, Netherlands; 6Department of Urology, University Medical Center, Rotterdam, Netherlands; 7Department of Biology, The Bioinformatics Centre, University of Copenhagen, Copenhagen, Denmark; 8The Novo Nordisk Center for Stem Cell Biology, Faculty of Health and Medical Sciences University of Copenhagen, Copenhagen, Denmark; 9Cell Biology Program, Memorial Sloan Kettering Cancer Center, New York, United States; 10Center for Epigenetics Research, Memorial Sloan Kettering Cancer Center, New York, United States *For correspondence: [email protected]; Abstract The PLZF transcription factor is essential for osteogenic differentiation of hMSCs; [email protected] (SAS); however, its regulation and molecular function during this process is not fully understood. Here, we [email protected] (KHA) revealed that the ZBTB16 locus encoding PLZF, is repressed by Polycomb (PcG) and H3K27me3 in Competing interests: The naive hMSCs. At the pre-osteoblast stage of differentiation, the locus lost PcG binding and authors declare that no H3K27me3, gained JMJD3 recruitment, and H3K27ac resulting in high expression of PLZF. -
Mechanisms of Salmonella Attachment and Survival on In-Shell Black Peppercorns, Almonds, and Hazelnuts
UC Irvine UC Irvine Previously Published Works Title Mechanisms of Salmonella Attachment and Survival on In-Shell Black Peppercorns, Almonds, and Hazelnuts. Permalink https://escholarship.org/uc/item/5534264q Authors Li, Ye Salazar, Joelle K He, Yingshu et al. Publication Date 2020 DOI 10.3389/fmicb.2020.582202 Peer reviewed eScholarship.org Powered by the California Digital Library University of California fmicb-11-582202 October 19, 2020 Time: 10:46 # 1 ORIGINAL RESEARCH published: 23 October 2020 doi: 10.3389/fmicb.2020.582202 Mechanisms of Salmonella Attachment and Survival on In-Shell Black Peppercorns, Almonds, and Hazelnuts Ye Li1, Joelle K. Salazar2, Yingshu He1, Prerak Desai3, Steffen Porwollik3, Weiping Chu3, Palma-Salgado Sindy Paola4, Mary Lou Tortorello2, Oscar Juarez5, Hao Feng4, Michael McClelland3* and Wei Zhang1* 1 Department of Food Science and Nutrition, Illinois Institute of Technology, Bedford Park, IL, United States, 2 Division of Food Processing Science and Technology, U.S. Food and Drug Administration, Bedford Park, IL, United States, 3 Department of Microbiology and Molecular Genetics, University of California, Irvine, Irvine, CA, United States, 4 Department of Food Science and Human Nutrition, University of Illinois at Urbana-Champaign, Urbana, IL, United States, 5 Department Edited by: of Biology, Illinois Institute of Technology, Chicago, IL, United States Chrysoula C. Tassou, Institute of Technology of Agricultural Products, Hellenic Agricultural Salmonella enterica subspecies I (ssp 1) is the leading cause of hospitalizations and Organization, Greece deaths due to known bacterial foodborne pathogens in the United States and is Reviewed by: frequently implicated in foodborne disease outbreaks associated with spices and nuts. -
Exoribonuclease Nibbler Shapes the 3″ Ends of Micrornas
Current Biology 21, 1878–1887, November 22, 2011 ª2011 Elsevier Ltd All rights reserved DOI 10.1016/j.cub.2011.09.034 Article The 30-to-50 Exoribonuclease Nibbler Shapes the 30 Ends of MicroRNAs Bound to Drosophila Argonaute1 Bo W. Han,1 Jui-Hung Hung,2 Zhiping Weng,2 precursor miRNAs (pre-miRNAs) [8]. Pre-miRNAs comprise Phillip D. Zamore,1,* and Stefan L. Ameres1,* a single-stranded loop and a partially base-paired stem whose 1Howard Hughes Medical Institute and Department of termini bear the hallmarks of RNase III processing: a two-nucle- Biochemistry and Molecular Pharmacology otide 30 overhang, a 50 phosphate, and a 30 hydroxyl group. 2Program in Bioinformatics and Integrative Biology Nuclear pre-miRNAs are exported by Exportin 5 to the cyto- University of Massachusetts Medical School, plasm, where the RNase III enzyme Dicer liberates w22 nt 364 Plantation Street, Worcester, MA 01605, USA mature miRNA/miRNA* duplexes from the pre-miRNA stem [9–12]. Like all Dicer products, miRNA duplexes contain two- nucleotide 30 overhangs, 50 phosphate, and 30 hydroxyl groups. Summary In flies, Dicer-1 cleaves pre-miRNAs to miRNAs, whereas Dicer-2 converts long double-stranded RNA (dsRNA) into Background: MicroRNAs (miRNAs) are w22 nucleotide (nt) small interfering RNAs (siRNAs), which direct RNA interference small RNAs that control development, physiology, and pathol- (RNAi), a distinct small RNA silencing pathway required for ogy in animals and plants. Production of miRNAs involves the host defense against viral infection and somatic transposon sequential processing of primary hairpin-containing RNA poly- mobilization, as well as gene silencing triggered by exogenous merase II transcripts by the RNase III enzymes Drosha in the dsRNA [13, 14]. -
Supplementary Materials
Supplementary Materials COMPARATIVE ANALYSIS OF THE TRANSCRIPTOME, PROTEOME AND miRNA PROFILE OF KUPFFER CELLS AND MONOCYTES Andrey Elchaninov1,3*, Anastasiya Lokhonina1,3, Maria Nikitina2, Polina Vishnyakova1,3, Andrey Makarov1, Irina Arutyunyan1, Anastasiya Poltavets1, Evgeniya Kananykhina2, Sergey Kovalchuk4, Evgeny Karpulevich5,6, Galina Bolshakova2, Gennady Sukhikh1, Timur Fatkhudinov2,3 1 Laboratory of Regenerative Medicine, National Medical Research Center for Obstetrics, Gynecology and Perinatology Named after Academician V.I. Kulakov of Ministry of Healthcare of Russian Federation, Moscow, Russia 2 Laboratory of Growth and Development, Scientific Research Institute of Human Morphology, Moscow, Russia 3 Histology Department, Medical Institute, Peoples' Friendship University of Russia, Moscow, Russia 4 Laboratory of Bioinformatic methods for Combinatorial Chemistry and Biology, Shemyakin-Ovchinnikov Institute of Bioorganic Chemistry of the Russian Academy of Sciences, Moscow, Russia 5 Information Systems Department, Ivannikov Institute for System Programming of the Russian Academy of Sciences, Moscow, Russia 6 Genome Engineering Laboratory, Moscow Institute of Physics and Technology, Dolgoprudny, Moscow Region, Russia Figure S1. Flow cytometry analysis of unsorted blood sample. Representative forward, side scattering and histogram are shown. The proportions of negative cells were determined in relation to the isotype controls. The percentages of positive cells are indicated. The blue curve corresponds to the isotype control. Figure S2. Flow cytometry analysis of unsorted liver stromal cells. Representative forward, side scattering and histogram are shown. The proportions of negative cells were determined in relation to the isotype controls. The percentages of positive cells are indicated. The blue curve corresponds to the isotype control. Figure S3. MiRNAs expression analysis in monocytes and Kupffer cells. Full-length of heatmaps are presented. -
Mir-17-92 Fine-Tunes MYC Expression and Function to Ensure
ARTICLE Received 31 Mar 2015 | Accepted 22 Sep 2015 | Published 10 Nov 2015 DOI: 10.1038/ncomms9725 OPEN miR-17-92 fine-tunes MYC expression and function to ensure optimal B cell lymphoma growth Marija Mihailovich1, Michael Bremang1, Valeria Spadotto1, Daniele Musiani1, Elena Vitale1, Gabriele Varano2,w, Federico Zambelli3, Francesco M. Mancuso1,w, David A. Cairns1,w, Giulio Pavesi3, Stefano Casola2 & Tiziana Bonaldi1 The synergism between c-MYC and miR-17-19b, a truncated version of the miR-17-92 cluster, is well-documented during tumor initiation. However, little is known about miR-17-19b function in established cancers. Here we investigate the role of miR-17-19b in c-MYC-driven lymphomas by integrating SILAC-based quantitative proteomics, transcriptomics and 30 untranslated region (UTR) analysis upon miR-17-19b overexpression. We identify over one hundred miR-17-19b targets, of which 40% are co-regulated by c-MYC. Downregulation of a new miR-17/20 target, checkpoint kinase 2 (Chek2), increases the recruitment of HuR to c- MYC transcripts, resulting in the inhibition of c-MYC translation and thus interfering with in vivo tumor growth. Hence, in established lymphomas, miR-17-19b fine-tunes c-MYC activity through a tight control of its function and expression, ultimately ensuring cancer cell homeostasis. Our data highlight the plasticity of miRNA function, reflecting changes in the mRNA landscape and 30 UTR shortening at different stages of tumorigenesis. 1 Department of Experimental Oncology, European Institute of Oncology, Via Adamello 16, Milan 20139, Italy. 2 Units of Genetics of B cells and lymphomas, IFOM, FIRC Institute of Molecular Oncology Foundation, Milan 20139, Italy. -
Aneuploidy: Using Genetic Instability to Preserve a Haploid Genome?
Health Science Campus FINAL APPROVAL OF DISSERTATION Doctor of Philosophy in Biomedical Science (Cancer Biology) Aneuploidy: Using genetic instability to preserve a haploid genome? Submitted by: Ramona Ramdath In partial fulfillment of the requirements for the degree of Doctor of Philosophy in Biomedical Science Examination Committee Signature/Date Major Advisor: David Allison, M.D., Ph.D. Academic James Trempe, Ph.D. Advisory Committee: David Giovanucci, Ph.D. Randall Ruch, Ph.D. Ronald Mellgren, Ph.D. Senior Associate Dean College of Graduate Studies Michael S. Bisesi, Ph.D. Date of Defense: April 10, 2009 Aneuploidy: Using genetic instability to preserve a haploid genome? Ramona Ramdath University of Toledo, Health Science Campus 2009 Dedication I dedicate this dissertation to my grandfather who died of lung cancer two years ago, but who always instilled in us the value and importance of education. And to my mom and sister, both of whom have been pillars of support and stimulating conversations. To my sister, Rehanna, especially- I hope this inspires you to achieve all that you want to in life, academically and otherwise. ii Acknowledgements As we go through these academic journeys, there are so many along the way that make an impact not only on our work, but on our lives as well, and I would like to say a heartfelt thank you to all of those people: My Committee members- Dr. James Trempe, Dr. David Giovanucchi, Dr. Ronald Mellgren and Dr. Randall Ruch for their guidance, suggestions, support and confidence in me. My major advisor- Dr. David Allison, for his constructive criticism and positive reinforcement. -
Paternal Finasteride Treatment Can Influence the Testicular
Article Paternal Finasteride Treatment Can Influence the Testicular Transcriptome Profile of Male Offspring—Preliminary Study Agnieszka Kolasa 1,* , Dorota Rogi ´nska 2 , Sylwia Rzeszotek 1 , Bogusław Machali ´nski 2 and Barbara Wiszniewska 1 1 Department of Histology and Embryology, Pomeranian Medical University (PMU), Powsta´nców Wlkp. 72 Avene, 70-111 Szczecin, Poland; [email protected] (S.R.); [email protected] (B.W.) 2 Department of General Pathology, Pomeranian Medical University, Powsta´nców Wlkp. 72 Avene, 70-111 Szczecin, Poland; [email protected] (D.R.); [email protected] (B.M.) * Correspondence: [email protected]; Tel.: +48-91-466-16-77 Abstract: (1) Background: Hormone-dependent events that occur throughout spermatogenesis during postnatal testis maturation are significant for adult male fertility. Any disturbances in the T/DHT ratio in male progeny born from females fertilized by finasteride-treated male rats (F0:Fin) can result in the impairment of testicular physiology. The goal of this work was to profile the testicular transcriptome in the male filial generation (F1:Fin) from paternal F0:Fin rats. (2) Methods: The subject material for the study were testis from immature and mature male rats born from females fertilized by finasteride-treated rats. Testicular tissues from the offspring were used in microarray analyses. (3) Results: The top 10 genes having the highest and lowest fold change values were mainly those that encoded odoriferous (Olfr: 31, 331, 365, 633, 774, 814, 890, 935, 1109, 1112, 1173, 1251, 1259, 1253, 1383) Citation: Kolasa, A.; Rogi´nska,D.; Vmn1r 50 103 210 211 Vmn2r 3 23 99 RIKEN cDNA 5430402E10 Rzeszotek, S.; Machali´nski,B.; and vomeronasal ( : , , , ; : , , ) receptors and , Wiszniewska, B. -
Characterization of the Mammalian RNA Exonuclease 5/NEF-Sp As a Testis-Specific Nuclear 3′′′′′ → 5′′′′′ Exoribonuclease
Downloaded from rnajournal.cshlp.org on October 7, 2021 - Published by Cold Spring Harbor Laboratory Press Characterization of the mammalian RNA exonuclease 5/NEF-sp as a testis-specific nuclear 3′′′′′ → 5′′′′′ exoribonuclease SARA SILVA,1,2 DAVID HOMOLKA,1 and RAMESH S. PILLAI1 1Department of Molecular Biology, University of Geneva, CH-1211 Geneva 4, Switzerland 2European Molecular Biology Laboratory, Grenoble Outstation, 38042, France ABSTRACT Ribonucleases catalyze maturation of functional RNAs or mediate degradation of cellular transcripts, activities that are critical for gene expression control. Here we identify a previously uncharacterized mammalian nuclease family member NEF-sp (RNA exonuclease 5 [REXO5] or LOC81691) as a testis-specific factor. Recombinant human NEF-sp demonstrates a divalent metal ion-dependent 3′′′′′ → 5′′′′′ exoribonuclease activity. This activity is specific to single-stranded RNA substrates and is independent of their length. The presence of a 2′′′′′-O-methyl modification at the 3′′′′′ end of the RNA substrate is inhibitory. Ectopically expressed NEF-sp localizes to the nucleolar/nuclear compartment in mammalian cell cultures and this is dependent on an amino-terminal nuclear localization signal. Finally, mice lacking NEF-sp are viable and display normal fertility, likely indicating overlapping functions with other nucleases. Taken together, our study provides the first biochemical and genetic exploration of the role of the NEF-sp exoribonuclease in the mammalian genome. Keywords: NEF-sp; LOC81691; Q96IC2; REXON; RNA exonuclease 5; REXO5; 2610020H08Rik INTRODUCTION clease-mediated processing to create their final 3′ ends: poly(A) tails of most mRNAs or the hairpin structure of Spermatogenesis is the process by which sperm cells are replication-dependent histone mRNAs (Colgan and Manley produced in the male germline. -
BRCA1 Binds TERRA RNA and Suppresses R-Loop-Based Telomeric DNA Damage ✉ Jekaterina Vohhodina 1,2 , Liana J
ARTICLE https://doi.org/10.1038/s41467-021-23716-6 OPEN BRCA1 binds TERRA RNA and suppresses R-Loop-based telomeric DNA damage ✉ Jekaterina Vohhodina 1,2 , Liana J. Goehring1, Ben Liu1,2, Qing Kong1,2, Vladimir V. Botchkarev Jr.1,2, Mai Huynh1, Zhiqi Liu1, Fieda O. Abderazzaq1,2, Allison P. Clark1,2, Scott B. Ficarro1,3,4,5, Jarrod A. Marto 1,3,4,5, ✉ Elodie Hatchi 1,2 & David M. Livingston 1,2 R-loop structures act as modulators of physiological processes such as transcription termi- 1234567890():,; nation, gene regulation, and DNA repair. However, they can cause transcription-replication conflicts and give rise to genomic instability, particularly at telomeres, which are prone to forming DNA secondary structures. Here, we demonstrate that BRCA1 binds TERRA RNA, directly and physically via its N-terminal nuclear localization sequence, as well as telomere- specific shelterin proteins in an R-loop-, and a cell cycle-dependent manner. R-loop-driven BRCA1 binding to CpG-rich TERRA promoters represses TERRA transcription, prevents TERRA R-loop-associated damage, and promotes its repair, likely in association with SETX and XRN2. BRCA1 depletion upregulates TERRA expression, leading to overly abundant TERRA R-loops, telomeric replication stress, and signs of telomeric aberrancy. Moreover, BRCA1 mutations within the TERRA-binding region lead to an excess of TERRA-associated R- loops and telomeric abnormalities. Thus, normal BRCA1/TERRA binding suppresses telomere-centered genome instability. 1 Department of Cancer Biology, Dana-Farber Cancer Institute, Boston, MA, USA. 2 Department of Genetics, Harvard Medical School, Boston, MA, USA. 3 Blais Proteomics Center, Dana-Farber Cancer Institute, Boston, MA, USA. -
Upregulation in Pregnancy Cells Correlates with IL-10 and Bcl-2
Published June 3, 2013, doi:10.4049/jimmunol.1203165 The Journal of Immunology Fetal–Maternal Alignment of Regulatory T Cells Correlates with IL-10 and Bcl-2 Upregulation in Pregnancy Brigitte Santner-Nanan,*,1 Kathrin Straubinger,†,1 Peter Hsu,*,‡,1 Grant Parnell,* Ben Tang,* Bei Xu,x Angela Makris,x Annemarie Hennessy,x,{ Michael J. Peek,* Dirk H. Busch,†,‖ Clarissa Prazeres da Costa,† and Ralph Nanan* Transplacental immune regulation refers to the concept that during pregnancy, significant cross-talk occurs between the maternal and fetal immune system with potential long-term effects for both the mother and child. In this study, we made the surprising observation that there is a strong correlation of peripheral blood regulatory T (Treg) cells between the mother and the fetus. In contrast, there is no significant Treg cell correlation between paternal fetal dyads (pairs), suggesting that the specific context of pregnancy, rather than the genetic parental similarity to the fetus, is responsible for this correlation. Gene microarray analysis of Treg cells identified a typical IL-10–dependent signature in maternal and fetal Treg cells. In addition, a direct correlation of serum IL-10 protein levels between maternal fetal dyads was observed. Furthermore, we show that maternal serum IL-10 levels correlate with serum estradiol and estriol, implicating hormonal involvement in this alignment. Interestingly, we show that Treg cells possess higher expression of IL-10 receptor a and that Treg cell IL-10 receptor a expression directly correlates with their Bcl-2 expression. Indeed, in vitro data in both humans and mice demonstrate that IL-10 upregulates Bcl-2 specifically in Treg cells but not non-Treg cells. -
Role for Cytoplasmic Nucleotide Hydrolysis in Hepatic Function and Protein Synthesis
Role for cytoplasmic nucleotide hydrolysis in hepatic function and protein synthesis Benjamin H. Hudson1, Joshua P. Frederick1, Li Yin Drake, Louis C. Megosh, Ryan P. Irving, and John D. York2,3 Department of Pharmacology and Cancer Biology, Howard Hughes Medical Institute, Duke University Medical Center, Durham, NC 27710 Edited by David W. Russell, University of Texas Southwestern Medical Center, Dallas, TX, and approved February 11, 2013 (received for review March 26, 2012) Nucleotide hydrolysis is essential for many aspects of cellular (5–7), which is degraded to 5′-AMP by a family of enzymes known function. In the case of 3′,5′-bisphosphorylated nucleotides, mam- as 3′-nucleotidases (8). mals possess two related 3′-nucleotidases, Golgi-resident 3′-phos- Mammalian genomes encode two 3′-nucleotidases, the recently phoadenosine 5′-phosphate (PAP) phosphatase (gPAPP) and characterized Golgi-resident PAP phosphatase (gPAPP) and Bisphosphate 3′-nucleotidase 1 (Bpnt1). gPAPP and Bpnt1 localize Bisphosphate 3′-nucleotidase 1 (Bpnt1), which localize to the to distinct subcellular compartments and are members of a con- Golgi lumen and cytoplasm, respectively (Fig. 1B)(8–11). gPAPP served family of metal-dependent lithium-sensitive enzymes. Al- and Bpnt1 are members of a family of small-molecule phospha- though recent studies have demonstrated the importance of tases whose activities are both dependent on divalent cations and gPAPP for proper skeletal development in mice and humans, the inhibited by lithium (8). The family comprises seven mammalian role of Bpnt1 in mammals remains largely unknown. Here we re- gene products: fructose bisphosphatase 1 and 2, inositol monophos- port that mice deficient for Bpnt1 do not exhibit skeletal defects phatase 1 and 2, inositol polyphosphate 1-phosphatase, gPAPP, but instead develop severe liver pathologies, including hypopro- and Bpnt1 (Fig.