Laboratory Experiments on Speciation
Total Page:16
File Type:pdf, Size:1020Kb
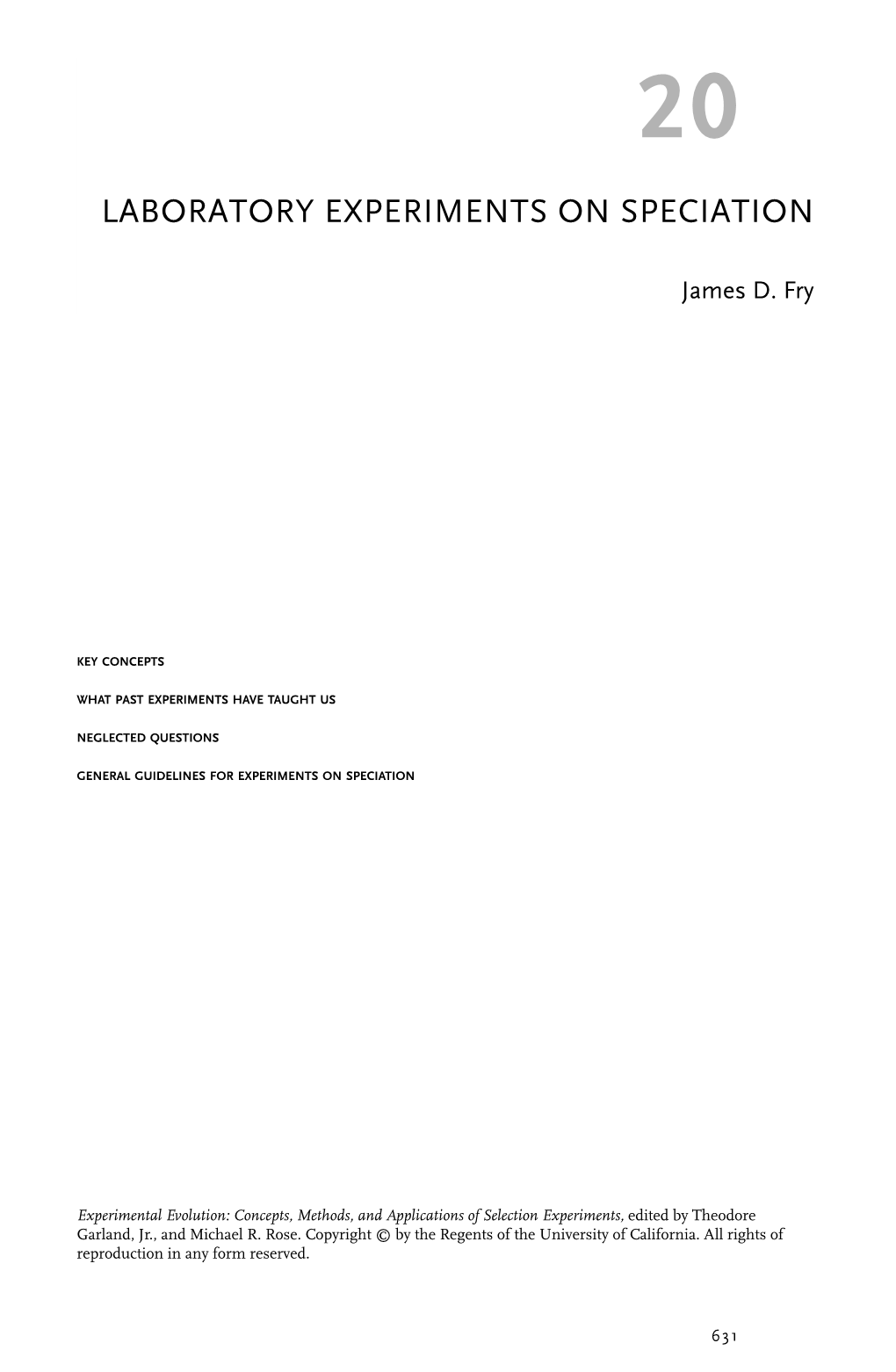
Load more
Recommended publications
-
Sympatric Speciation: Models and Empirical Evidence
ANRV328-ES38-19 ARI 24 September 2007 7:20 Sympatric Speciation: Models and Empirical Evidence Daniel I. Bolnick1 and Benjamin M. Fitzpatrick2 1Section of Integrative Biology, University of Texas, Austin, Texas 78712; email: [email protected] 2Department of Ecology and Evolutionary Biology, University of Tennessee, Knoxville, Tennessee 37996; email: benfi[email protected] Annu. Rev. Ecol. Evol. Syst. 2007. 38:459–87 Key Words First published online as a Review in Advance on assortative mating, disruptive selection, reinforcement August 8, 2007 reproductive isolation The Annual Review of Ecology, Evolution, and Systematics is online at Abstract http://ecolsys.annualreviews.org Sympatric speciation, the evolution of reproductive isolation with- This article’s doi: 10.1146/annurev.ecolsys.38.091206.095804 out geographic barriers, remains highly contentious. As a result of new empirical examples and theory, it is now generally accepted that Copyright c 2007 by Annual Reviews. All rights reserved sympatric speciation has occurred in at least a few instances, and is theoretically plausible. Instead, debate has shifted to whether sym- by Rutgers University Libraries on 09/21/09. For personal use only. 1543-592X/07/1201-0459$20.00 patric speciation is common, and whether models’ assumptions are generally met in nature. The relative frequency of sympatric spe- ciation will be difficult to resolve, because biogeographic changes have obscured geographical patterns underlying many past specia- Annu. Rev. Ecol. Evol. Syst. 2007.38:459-487. Downloaded from arjournals.annualreviews.org tion events. In contrast, progress is being made on evaluating the empirical validity of key theoretical conditions for sympatric spe- ciation. Disruptive selection and direct selection on mating traits, which should facilitate sympatric speciation, are biologically well supported. -
Microevolution and the Genetics of Populations Microevolution Refers to Varieties Within a Given Type
Chapter 8: Evolution Lesson 8.3: Microevolution and the Genetics of Populations Microevolution refers to varieties within a given type. Change happens within a group, but the descendant is clearly of the same type as the ancestor. This might better be called variation, or adaptation, but the changes are "horizontal" in effect, not "vertical." Such changes might be accomplished by "natural selection," in which a trait within the present variety is selected as the best for a given set of conditions, or accomplished by "artificial selection," such as when dog breeders produce a new breed of dog. Lesson Objectives ● Distinguish what is microevolution and how it affects changes in populations. ● Define gene pool, and explain how to calculate allele frequencies. ● State the Hardy-Weinberg theorem ● Identify the five forces of evolution. Vocabulary ● adaptive radiation ● gene pool ● migration ● allele frequency ● genetic drift ● mutation ● artificial selection ● Hardy-Weinberg theorem ● natural selection ● directional selection ● macroevolution ● population genetics ● disruptive selection ● microevolution ● stabilizing selection ● gene flow Introduction Darwin knew that heritable variations are needed for evolution to occur. However, he knew nothing about Mendel’s laws of genetics. Mendel’s laws were rediscovered in the early 1900s. Only then could scientists fully understand the process of evolution. Microevolution is how individual traits within a population change over time. In order for a population to change, some things must be assumed to be true. In other words, there must be some sort of process happening that causes microevolution. The five ways alleles within a population change over time are natural selection, migration (gene flow), mating, mutations, or genetic drift. -
Experimental Evolution
Heredity (2008) 100, 441–442 & 2008 Nature Publishing Group All rights reserved 0018-067X/08 $30.00 www.nature.com/hdy EDITORIAL Experimental evolution Heredity (2008) 100, 441–442; doi:10.1038/hdy.2008.19 and their interactions, it should be possible to predict which genes will be altered, how they will be altered and in what order, in any defined environment. Our under- Microcosms are used in ecology and evolution to shrink standing of cell biology is as yet wholly inadequate for space and time to manageable scales. They have never the task, but the smaller genomes of viruses might be been extensively used in ecology, because many ecolo- more accessible. Bull and Molineux describe the most gists doubt that any important features of large complex ambitious attempts to date in pursuit of this grail, using systems, such as lakes, can be profitably investigated in phage T7 of E. coli. Given the detailed knowledge of T7 small chambers or vials (Carpenter, 1996). Consequently, genetics, it should be possible to introduce a specific ecology lacks any generally accepted model system genetic lesion and then predict what compensatory to facilitate the cumulative growth of understanding of mutations will be fixed so as to restore the lost function. how communities work. Evolutionary biologists have In many cases, these mutations were correctly identified been less skeptical, and at least three major schools and for very simple genomes, at least, a nearly complete have adopted microcosm methods. Perhaps the best understanding of adaptation seems to be within grasp. known is the extensive exploration of the effects of artificial selection in Drosophila (and to a lesser extent in At the same time, both Ferenci and Bull and Molineux other organisms, such as mice). -
Genetic Structure and Eco-Geographical Differentiation of Lancea Tibetica in the Qinghai-Tibetan Plateau
G C A T T A C G G C A T genes Article Genetic Structure and Eco-Geographical Differentiation of Lancea tibetica in the Qinghai-Tibetan Plateau Xiaofeng Chi 1,2 , Faqi Zhang 1,2,* , Qingbo Gao 1,2, Rui Xing 1,2 and Shilong Chen 1,2,* 1 Key Laboratory of Adaptation and Evolution of Plateau Biota, Northwest Institute of Plateau Biology, Chinese Academy of Sciences, Xining 810001, China; [email protected] (X.C.); [email protected] (Q.G.); [email protected] (R.X.) 2 Qinghai Provincial Key Laboratory of Crop Molecular Breeding, Xining 810001, China * Correspondence: [email protected] (F.Z.); [email protected] (S.C.) Received: 14 December 2018; Accepted: 24 January 2019; Published: 29 January 2019 Abstract: The uplift of the Qinghai-Tibetan Plateau (QTP) had a profound impact on the plant speciation rate and genetic diversity. High genetic diversity ensures that species can survive and adapt in the face of geographical and environmental changes. The Tanggula Mountains, located in the central of the QTP, have unique geographical significance. The aim of this study was to investigate the effect of the Tanggula Mountains as a geographical barrier on plant genetic diversity and structure by using Lancea tibetica. A total of 456 individuals from 31 populations were analyzed using eight pairs of microsatellite makers. The total number of alleles was 55 and the number per locus ranged from 3 to 11 with an average of 6.875. The polymorphism information content (PIC) values ranged from 0.2693 to 0.7761 with an average of 0.4378 indicating that the eight microsatellite makers were efficient for distinguishing genotypes. -
Allopatric Speciation with Little Niche Divergence Is Common Among
Journal of Biogeography (J. Biogeogr.) (2016) 43, 591–602 ORIGINAL Allopatric speciation with little niche ARTICLE divergence is common among alpine Primulaceae Florian C. Boucher1*, Niklaus E. Zimmermann2,3 and Elena Conti1 1Institute of Systematic Botany, University of ABSTRACT Zurich,€ 8008 Zurich,€ Switzerland, 2Dynamic Aim Despite the accumulation of cases describing fast radiations of alpine Macroecology, Swiss Federal Research plants, we still have limited understanding of the drivers of speciation in alpine Institute WSL, 8903 Birmensdorf, Switzerland, 3Department of Environmental floras and of the precise the timing of their diversification. Here, we investi- Systems Science, Swiss Federal Institute of gated spatial and temporal patterns of speciation in three groups of alpine Technology ETH, CH-8092 Zurich,€ Primulaceae. Switzerland Location Mountains of the European Alpine System. Methods We built a new phylogeny of Primulaceae including all species in three focal groups: Androsace sect. Aretia, Primula sect. Auricula and Soldanella. Combining phylogenetic information with a detailed climatic data set, we investigated patterns of range and ecological overlap between sister-species using an approach that takes phylogenetic uncertainty into account. Finally, we investigated temporal trajectories of diversification in the three focal groups. Results We found that a large majority of sister-species pairs in the three groups are strictly allopatric and show little differences in substrate and cli- matic preferences, a result that was robust to phylogenetic uncertainty. While rates of diversification have remained constant in Soldanella, both Androsace sect. Aretia and Primula sect. Auricula showed decreased diversification rates in the Pleistocene compared to previous geological epochs. Main conclusions Allopatric speciation with little niche divergence appears to have been by far the most common mode of speciation across the three groups studied. -
Species Change Over Time
KEY CONCEPT Species change over time. BEFORE, you learned NOW, you will learn • Fossils are evidence of earlier • About early ideas and observa- life tions on evolution • More complex organisms have • How Darwin developed his developed over time theory of natural selection • Mass extinctions contributed to • How new species arise from the development of Earth’s older species history VOCABULARY THINK ABOUT evolution p. 797 How have telephones changed over time? natural selection p. 801 adaptation p. 802 Today people across the world can speciation p. 804 communicate in many different ways. One of the most common ways is over the telephone. Looking at the two pictures, can you describe how this form of communication has changed over time? Scientists explore the concept of evolution. MAIN IDEA AND DETAILS In a general sense, evolution involves a change over time. You could Make a chart for the main say that the way humans communicate has evolved. Certainly idea scientists explore the concept of evolution. telephones have changed over time. The first telephones were the size Include details about scien- of a shoebox. Today a telephone can fit in the palm of your hand and tists’ observations. can send images as well as sound. In biology,evolution refers to the process though which species change over time. The change results from a change in the genetic material of an organism and is passed from one generation to the next. Check Your Reading What is evolution? Chapter 23: History of Life 797 Early Ideas reading tip In the early 1800s, a French scientist named Jean Baptiste de Lamarck The word acquire comes was the first scientist to propose a model of how life evolves. -
Speciation in Geographical Setting
Speciation Speciation 2019 2019 The degree of reproductive isolation Substantial variation exists in among geographical sets of species - anagenesis populations within an actively 1859 1859 evolving species complex is often Achillea - yarrow tested by crossing experiments — as in the tidy tips of California 100K bp 100K bp back in time back in time back Rubus parviforus K = 4 mean population assignment 2 mya 2 mya ID CO WI_Door 5 mya BC_Hixton BC_MtRob WI_BruleS 5 mya BC_McLeod CA_Klamath MI_Windigo WA_Cascade WA_BridgeCr SD_BlackHills OR_Willamette MI_Drummond Speciation Speciation 2019 Reproductive isolation will ultimately stop all Although simple in concept, the recognition of species and thus the definition genetic connections among sets of populations of what are species have been controversial — more than likely due to the – cladogenesis or speciation continuum nature of the pattern resulting from the process of speciation 1859 Example: mechanical isolation via floral shape changes and pollinators between two parapatric species of California Salvia (sage) 100K bp back in time back 2 mya S. mellifera 5 mya Salvia apiana 1 Speciation Speciation Although simple in concept, the recognition of species and thus the definition Animal examples of speciation often show of what are species have been controversial — more than likely due to the clear reproductive barriers - hence zoologists continuum nature of the pattern resulting from the process of speciation preference (as opposed to botanists) for the Reproductive isolating Biological -
Long-Term Experimental Evolution in Escherichia Coli. V. Effects of Recombination with Immigrant Genotypes on the Rate of Bacterial Evolution
© Birkhliuser Verlag, Basel, 1997 J. evol. bioi. 10 (1997) 743-769 1010-061X/97/050743-27 $ 1.50 + 0.20/0 I Journal of Evolutionary Biology Long-term experimental evolution in Escherichia coli. V. Effects of recombination with immigrant genotypes on the rate of bacterial evolution 1 2 3 V. Souza, P. E. Turner • and R. E. LenskF·* 1Centro de Ecologia, Universidad Nacional Aut6noma de Mexico, Coyoacim, Ciudad de Mexico, 04510, Mexico 2 Center for Microbial Ecology, Michigan State University, East Lansing MI 48824, USA 3 Current address: Department of Zoology, University of Maryland, College Park MD 20742, USA Key words: Complex selection; conjugation; Escherichia coli; experimental evolu tion; hitchhiking; migration; plasmids; recombination. Abstract This study builds upon an earlier experiment that examined the dynamics of mean fitness in evolving populations of Escherichia coli in which mutations were the sole source of genetic variation. During thousands of generations in a constant environment, the rate of improvement in mean fitness of these asexual populations slowed considerably from an initially rapid pace. In this study, we sought to determine whether sexual recombination with novel genotypes would reaccelerate the rate of adaption in these populations. To that end, treatment populations were propagated for an additional 1000 generations in the same environment as their ancestors, but they were periodically allowed to mate with an immigrant pool of genetically distinct Hfr (high frequency recombination) donors. These donors could transfer genes to the resident populations by conjugation, but the donors them selves could not grow in the experimental environment. Control populations were propagated under identical conditions, but in the absence of sexual recombination with the donors. -
Sympatric Speciation in Ants
When houseguests become parasites: Sympatric speciation in ants Stewart H. Berlocher* Department of Entomology, University of Illinois at Urbana–Champaign, 320 Morrill Hall, 505 South Goodwin Avenue, Urbana, IL 61801 pecies are part of the common related species, and we are finally, if still argue that sympatric speciation was not coinage of biology. Taxonomists dimly, seeing some of the specific genes limited to a handful of special cases, but name them, developmental biolo- that underlie reproductive isolation (3). was quite common. Bush was driven to gists deconstruct them, physiolo- But fundamental questions remain his stance by the observation that one S unanswered. A critical question is how gists compare them, ecologists count could find many groups of closely re- them, conservation biologists conserve reproductive isolation could evolve in lated, sympatric species that use differ- them, and evolutionary biologists study the face of gene flow; random mating ent ecological niches. Conspicuous their multiplication and extinction. It within a population and gene flow be- among these groups are parasites of ani- may be that only the individual is a tween neighboring populations are enor- mals and plants, which are often highly more important biological unit than the mously powerful homogenizing forces. specialized ecologically and frequently species. It is thus no surprise that Dar- How could selection tear a single popu- mate on the host, a factor that poten- win named his great work On the Origin lation into two reproductively isolated tially links any adaptation to a new host of Species, nor is it a surprise that many with a reduction in gene flow between evolutionary biologists today concen- the new and ancestral populations. -
Selection Experiments and Experimental Evolution of Performance and Physiology
Garland_ch12.qxd 8/3/09 2:03 PM Page 301 12 SELECTION EXPERIMENTS AND EXPERIMENTAL EVOLUTION OF PERFORMANCE AND PHYSIOLOGY John G. Swallow, Jack P. Hayes, Pawel Koteja, and Theodore Garland, Jr. THE IMPORTANCE OF REPLICATION Selection on VO2max and the Correlation between BMR and VO2max EXPERIMENTAL EVOLUTION OF MICE IN DIFFERENT THERMAL ENVIRONMENTS SEXUAL SELECTION: EFFECTS OF ORNAMENTS ON PERFORMANCE WIND TUNNEL FLIGHT IN DROSOPHILA Guppies ENDURANCE RUNNING AND STRESS-INDUCED ANALGESIA IN MICE Stalk-Eyed Flies ENDURANCE RUNNING IN RATS AND VOLUNTARY WHEEL “EXPERIMENTS” WITHOUT PRECISELY DEFINED SELECTION RUNNING IN MICE CRITERIA EVOLUTION OF THE RATE OF ENERGY METABOLISM Horse Racing IN RODENTS Greyhound Racing Selection on Basal Metabolic Rate PHYSIOLOGICAL DIFFERENCES AMONG STRAINS Selection on Heat Loss OF MICE AND BREEDS OF DOG Rate of Metabolism as a Hypothetical CONCLUSION Correlated Response Experimental Evolution: Concepts, Methods, and Applications of Selection Experiments, edited by Theodore Garland, Jr., and Michael R. Rose. Copyright © by the Regents of the University of California. All rights of reproduction in any form reserved. 301 Garland_ch12.qxd 8/3/09 2:03 PM Page 302 Since a seminal paper by Arnold (1983), direct measurement of whole-organism perfor- mance has become central to functional evolutionary biology (e.g., Arnold 2003; Ghalambor et al. 2003; Kingsolver and Huey 2003). In this context, “performance” can be most easily defined by example. Assuming that individuals can be fully motivated (e.g., see Swallow et al. 1998a; Harris and Steudel 2002; Losos et al. 2002; Tobalske et al. 2004), it is relatively easy to measure maximal sprint running speed of small mammals and lizards on photocell-timed racetracks or high-speed treadmills (e.g., Calsbeek and Irschick 2007; Chappell et al. -
Darwinian Evolution in a Translation-Coupled RNA Replication System Within a Cell-Like Compartment
ARTICLE Received 28 Mar 2013 | Accepted 22 Aug 2013 | Published 3 Oct 2013 DOI: 10.1038/ncomms3494 Darwinian evolution in a translation-coupled RNA replication system within a cell-like compartment Norikazu Ichihashi1,2, Kimihito Usui1, Yasuaki Kazuta1, Takeshi Sunami1,2, Tomoaki Matsuura1,2,3 & Tetsuya Yomo1,2,4 The ability to evolve is a key characteristic that distinguishes living things from non-living chemical compounds. The construction of an evolvable cell-like system entirely from non-living molecules has been a major challenge. Here we construct an evolvable artificial cell model from an assembly of biochemical molecules. The artificial cell model contains artificial genomic RNA that replicates through the translation of its encoded RNA replicase. We perform a long-term (600-generation) replication experiment using this system, in which mutations are spontaneously introduced into the RNA by replication error, and highly replicable mutants dominate the population according to Darwinian principles. During evolution, the genomic RNA gradually reinforces its interaction with the translated replicase, thereby acquiring competitiveness against selfish (parasitic) RNAs. This study provides the first experimental evidence that replicating systems can be developed through Darwinian evolution in a cell-like compartment, even in the presence of parasitic replicators. 1 Exploratory Research for Advanced Technology, Japan Science and Technology Agency, Osaka University, Suita, Osaka 565-0871, Japan. 2 Graduate School of Information Science and Technology, Osaka University, Suita, Osaka 565-0871, Japan. 3 Graduate School of Engineering, Osaka University, Suita, Osaka 565-0871, Japan. 4 Graduate School of Frontier Biosciences, Osaka University, Suita, Osaka 565-0871, Japan. Correspondence and requests for materials should be addressed to T.Y. -
Speciation and Recessive Mutations for BMC Biology
Essay: On the close relationship between speciation, inbreeding and recessive mutations. Etienne Joly, [email protected], Toulouse, September 2010 All the ideas developed in this essay are relatively simple, and most of them are related to many Foreword previously published works. So much work, however, This past year, 2009, was the Darwin year, celebrating has already been published on evolution and speciation the 200th anniversary of Charles Darwin's birth, and 150 that an autodidactic newcomer such as myself could years since the publication of his fabulous milestone not hope to read, let alone understand and remember all book, ‘The Origin of Species’ (to which I will the primary papers published previously on evolution subsequently refer to as ‘The Origin’). At the start of and speciation. If I have failed to acknowledge 2009, I was inhabited by a nagging ethical concern : how previous works developing ideas related to those put would humans deal with a situation where a group of forward here, the reader can be assured that this was individuals found themselves fertile among one another, not done maliciously but simply as a result of my but with limited fertility with the rest of the human race ? relative naivety on the subject. I do, however, hold the In other words, could speciation occur within the human firm conviction that, if some of the ideas developed in race ? This concern sprouted from the idea that this essay prove to be correct and relatively novel, it chromosomal rearrangements seemed to me like a very was only possible because of this naivety.