Importance of Biologically Active Aurora-Like Ultraviolet Emission: Stochastic Irradiation of Earth and Mars by Flares and Explosions
Total Page:16
File Type:pdf, Size:1020Kb
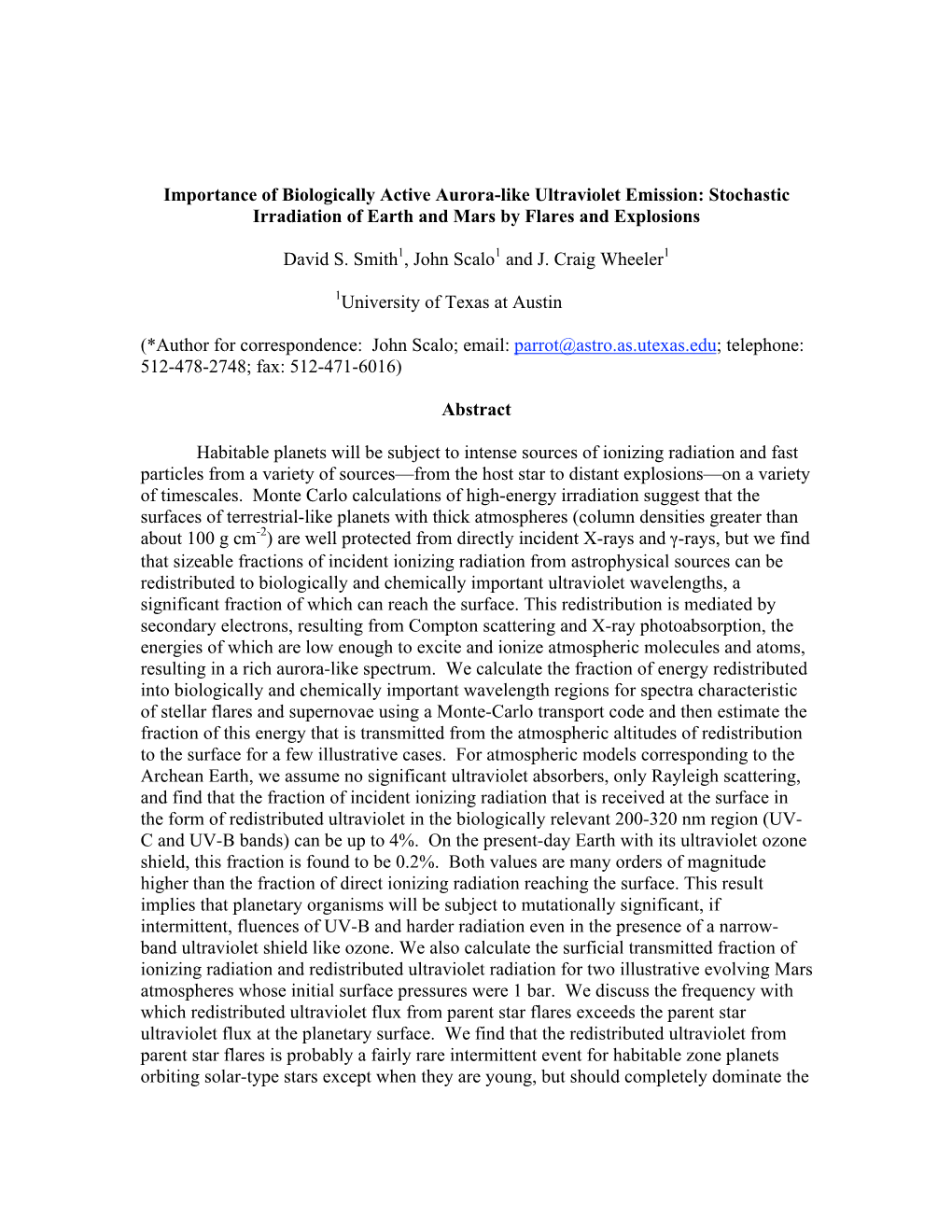
Load more
Recommended publications
-
Review of the State of Infrared Detectors for Astronomy in Retrospect of the June 2002 Workshop on Scientific Detectors for Astronomy
Review of the state of infrared detectors for astronomy in retrospect of the June 2002 Workshop on Scientific Detectors for Astronomy. Gert Fingera and James W. Beleticb a European Southern Obseravatory, D-85748 Garching, Germany b W. M. Keck Observatory 65-1120 Mamalahoa Hwy., Kamuela, Hi 96743, USA ABSTRACT Only two months ago, in June 2002, a workshop on scientific detectors for astronomy was held in Waimea, where for the first time both experts on optical CCD’s and infrared detectors working at the cutting edge of focal plane technology gathered. An overview of new developments in optical detectors such as CCD’s and CMOS devices will be given elsewhere in these proceedings [1]. This paper will focus on infrared detector developments carried out at the European Southern Observatory ESO and will also include selected highlights of infrared focal plane technology as presented at the Waimea workshop. Three main detector developments for ground based astronomers are currently pushing infrared focal plane technology. In the near infrared from 1 to 5 mm two technologies, both aiming for buttable 2Kx2K mosaics, will be reviewed, namely InSb and HgCdTe grown by LPE or MBE on Al2O3, Si or CdZnTe substrates. Blocked impurity band Si:As arrays cover the mid infrared spectral range from 8 to 28 mm. Since the video signal of infrared arrays, contrary to CCD’s, is DC coupled, long exposures with IR arrays are extremely susceptible to drifts and low frequency noise pick- up down to the mHz regime. New techniques to reduce thermal drifts and suppress low frequency noise with on-chip reference pixels will be discussed. -
High Frequency (HF)
Calhoun: The NPS Institutional Archive Theses and Dissertations Thesis Collection 1990-06 High Frequency (HF) radio signal amplitude characteristics, HF receiver site performance criteria, and expanding the dynamic range of HF digital new energy receivers by strong signal elimination Lott, Gus K., Jr. Monterey, California: Naval Postgraduate School http://hdl.handle.net/10945/34806 NPS62-90-006 NAVAL POSTGRADUATE SCHOOL Monterey, ,California DISSERTATION HIGH FREQUENCY (HF) RADIO SIGNAL AMPLITUDE CHARACTERISTICS, HF RECEIVER SITE PERFORMANCE CRITERIA, and EXPANDING THE DYNAMIC RANGE OF HF DIGITAL NEW ENERGY RECEIVERS BY STRONG SIGNAL ELIMINATION by Gus K. lott, Jr. June 1990 Dissertation Supervisor: Stephen Jauregui !)1!tmlmtmOlt tlMm!rJ to tJ.s. eave"ilIE'il Jlcg6iielw olil, 10 piolecl ailicallecl",olog't dU'ie 18S8. Btl,s, refttteste fer litis dOCdiii6i,1 i'lust be ,ele"ed to Sapeihil6iiddiil, 80de «Me, "aial Postg;aduulG Sclleel, MOli'CIG" S,e, 98918 &988 SF 8o'iUiid'ids" PM::; 'zt6lI44,Spawd"d t4aoal \\'&u 'al a a,Sloi,1S eai"i,al'~. 'Nsslal.;gtePl. Be 29S&B &198 .isthe 9aleMBe leclu,sicaf ,.,FO'iciaKe" 6alite., ea,.idiO'. Statio", AlexB •• d.is, VA. !!!eN 8'4!. ,;M.41148 'fl'is dUcO,.Mill W'ilai.,s aliilical data wlrose expo,l is idst,icted by tli6 Arlil! Eurse" SSPItial "at FRIis ee, 1:I.9.e. gec. ii'S1 sl. seq.) 01 tlls Exr;01l ftle!lIi"isllatioli Act 0' 19i'9, as 1tI'I'I0"e!ee!, "Filill ell, W.S.€'I ,0,,,,, 1i!4Q1, III: IIlIiI. 'o'iolatioils of ltrese expo,lla;;s ale subject to 960616 an.iudl pSiiaities. -
AN EMPIRICAL DETERMINATION of the INTERGALACTIC BACKGROUND LIGHT USING NEAR-INFRARED DEEP GALAXY SURVEY DATA out to 5 Μm and the GAMMA-RAY OPACITY of the UNIVERSE
The Astrophysical Journal, 784:138 (6pp), 2014 April 1 doi:10.1088/0004-637X/784/2/138 C 2014. The American Astronomical Society. All rights reserved. Printed in the U.S.A. AN EMPIRICAL DETERMINATION OF THE INTERGALACTIC BACKGROUND LIGHT USING NEAR-INFRARED DEEP GALAXY SURVEY DATA OUT TO 5 μm AND THE GAMMA-RAY OPACITY OF THE UNIVERSE Sean T. Scully1, Matthew A. Malkan2, and Floyd W. Stecker2,3 1 Department of Physics and Astronomy, James Madison University, Harrisonburg, VA 22807, USA 2 Department of Physics and Astronomy, University of California, Los Angeles, CA 90095, USA; [email protected] 3 Astrophysics Science Division, NASA/Goddard Space Flight Center, 8800 Greenbelt Road, Greenbelt, MD 20771, USA Received 2014 January 21; accepted 2014 February 24; published 2014 March 14 ABSTRACT We extend our previous model-independent determination of the intergalactic background light, based purely on galaxy survey data, out to a wavelength of 5 μm. Our approach enables us to constrain the range of photon densities, based on the uncertainties from observationally determined luminosity densities and colors. We further determine a 68% confidence upper and lower limit on the opacity of the universe to γ -rays up to energies of 1.6/(1 + z)TeV. A comparison of our lower limit redshift-dependent opacity curves to the opacity limits derived from the results of both ground-based air Cerenkov telescope and Fermi-LAT observations of PKS 1424+240 allows us to place a new upper limit on the redshift of this source, independent of IBL modeling. Key words: BL Lacertae objects: individual (PKS 1424+240) – diffuse radiation – gamma rays: general Online-only material: color figure <z< 1. -
Recent Studies on Hohlraum Energitics and Hohlraum Design
Recent studies on hohlraum energitics and hohlraum design Ke Lan, Wenyi Huo, Yongsheng Li, Guoli Ren, Xin Li, Xujun Meng, Changshu Wu, Siyang Zou, Xiumei Qiao, Peijun Gu, Wudi Zheng, Dongxian Lai, and Tinggui Feng Institute of Applied Physics and Computational Mathematics, P.O. Box 8009-14 Beijing, 100088, People’s Republic of China, E-mail: [email protected] Abstract In this paper, some recent studies on hohlraum physics at IAPCM (Institute of Applied Physics and Computational Mathematics) were presented, mainly including simulation study on hohlraum physics experiments on SGIII prototype, a novel method for simultaneously determining the maximum radiation temperature and M-band fraction inside a hohlraum, the design of Au + U + Au sandwich hohlraum for 300eV ignition target, and the way to give an initial design of hohlraum size and pertinent drive laser power in order to generate a required radiation profile. 1. Introduction Hohlraum plays a key role in the study of indirect drive inertial fusion, which converts the shaped laser pulse into a symmetrically time-dependent thermal radiation to assure a nearly isentropic compression of capsule to achieve thermonuclear ignition 1-6. Hence, worldwide IFE (Inertial Fusion Energy) scientists have been paying much efforts in the study of hohlraum physics 7-11, and a series of theory for hohlraum have been developed, such as the hohlraum coupling efficiency theory, capsule radiation uniformity theory 5, and plasma-filling model 12 . Inertial Fusion program began in China in 1993. We have laser facilities of SGII in Shanghai and SGIII prototype in Mianyang 13-15 . IAPCM in Beijing mainly engages in simulation and theoretical study of Inertial Fusion. -
The Effect of Gamma Radiation on the Conductivity of Sodium Chloride Michael Claudewell Jon Carlson Iowa State University
Iowa State University Capstones, Theses and Retrospective Theses and Dissertations Dissertations 1968 The effect of gamma radiation on the conductivity of sodium chloride Michael Claudewell Jon Carlson Iowa State University Follow this and additional works at: https://lib.dr.iastate.edu/rtd Part of the Nuclear Engineering Commons Recommended Citation Carlson, Michael Claudewell Jon, "The effect of gamma radiation on the conductivity of sodium chloride " (1968). Retrospective Theses and Dissertations. 3723. https://lib.dr.iastate.edu/rtd/3723 This Dissertation is brought to you for free and open access by the Iowa State University Capstones, Theses and Dissertations at Iowa State University Digital Repository. It has been accepted for inclusion in Retrospective Theses and Dissertations by an authorized administrator of Iowa State University Digital Repository. For more information, please contact [email protected]. This dissertation has been microiihned exactly as received 69-4221 CARLSON, Michael Claudewell Jon, 1939- THE EFFECT OF GAMMA RADIATION ON THE CONDUCTIVITY OF SODIUM CHLORIDE. Iowa State University, Ph.D., 1968 Engineering, nuclear University Microfilms, Inc., Ann Arbor, Michigan THE SFFECT OF GAMMA RADIATION ON THE CONDUCTIVITY OF SODIUM CHLORIDE by Michael Claudewell Jon Carlson A Dissertation Submitted to the Graduate Faculty in Partial Fulfillment of The Requirements for the Degree of DOCTOR OF PHILOSOPHY Major Subject: Nuclear Engineering Approved; Signature was redacted for privacy. Signature was redacted for privacy. -
Template BR Rec 2005.Dot
Report ITU-R M.2442-0 (11/2018) Current and future usage of railway radiocommunication systems between train and trackside M Series Mobile, radiodetermination, amateur and related satellite services ii Rep. ITU-R M.2442-0 Foreword The role of the Radiocommunication Sector is to ensure the rational, equitable, efficient and economical use of the radio- frequency spectrum by all radiocommunication services, including satellite services, and carry out studies without limit of frequency range on the basis of which Recommendations are adopted. The regulatory and policy functions of the Radiocommunication Sector are performed by World and Regional Radiocommunication Conferences and Radiocommunication Assemblies supported by Study Groups. Policy on Intellectual Property Right (IPR) ITU-R policy on IPR is described in the Common Patent Policy for ITU-T/ITU-R/ISO/IEC referenced in Resolution ITU-R 1. Forms to be used for the submission of patent statements and licensing declarations by patent holders are available from http://www.itu.int/ITU-R/go/patents/en where the Guidelines for Implementation of the Common Patent Policy for ITU-T/ITU-R/ISO/IEC and the ITU-R patent information database can also be found. Series of ITU-R Reports (Also available online at http://www.itu.int/publ/R-REP/en) Series Title BO Satellite delivery BR Recording for production, archival and play-out; film for television BS Broadcasting service (sound) BT Broadcasting service (television) F Fixed service M Mobile, radiodetermination, amateur and related satellite services P Radiowave propagation RA Radio astronomy RS Remote sensing systems S Fixed-satellite service SA Space applications and meteorology SF Frequency sharing and coordination between fixed-satellite and fixed service systems SM Spectrum management Note: This ITU-R Report was approved in English by the Study Group under the procedure detailed in Resolution ITU-R 1. -
Federal Communications Commission
Vol. 80 Thursday, No. 127 July 2, 2015 Part IV Federal Communications Commission 47 CFR Parts 2, 15, 80, 90, et al. WRC–12 Radiocommunication Conference (Geneva 2012); Proposed Rule VerDate Sep<11>2014 21:32 Jul 01, 2015 Jkt 235001 PO 00000 Frm 00001 Fmt 4717 Sfmt 4717 E:\FR\FM\02JYP2.SGM 02JYP2 asabaliauskas on DSK5VPTVN1PROD with PROPOSALS 38316 Federal Register / Vol. 80, No. 127 / Thursday, July 2, 2015 / Proposed Rules FEDERAL COMMUNICATIONS D Electronic Filers: Comments may be audio format), send an email to fcc504@ COMMISSION filed electronically using the Internet by fcc.gov or call the Consumer & accessing the ECFS: http:// Governmental Affairs Bureau at 202– 47 CFR Parts 2, 15, 80, 90, 97, and 101 fjallfoss.fcc.gov/ecfs2/. 418–0530 (voice), 202–418–0432 (tty). D Paper Filers: Parties that choose to [ET Docket No. 15–99; FCC 15–50] Summary of Notice of Proposed file by paper must file an original and Rulemaking WRC–12 Radiocommunication one copy of each filing. If more than one Conference (Geneva 2012) docket or rulemaking number appears in 1. In this Notice of Proposed the caption of this proceeding, filers Rulemaking (WRC–12 NPRM), the AGENCY: Federal Communications must submit two additional copies for Commission proposes to amend parts 2, Commission. each additional docket or rulemaking 15, 80, 90, 97, and 101 of its rules to ACTION: Proposed rule. number. implement allocation decisions from the D Filings can be sent by hand or Final Acts of the World SUMMARY: In this document, the messenger delivery, by commercial Radiocommunication Conference Commission proposes to implement overnight courier, or by first-class or (Geneva, 2012) (WRC–12 Final Acts) and certain allocation changes from the overnight U.S. -
Enhancement of Laser to X-Ray Conversion by a Double-Foil Gold Target E-Mail: [email protected] and [email protected]
IOP View metadata, citation and similar papers at core.ac.uk brought to you by CORE provided by Shanghai Institute of Optics and Fine Mechanics,Chinese Academy of Sciences Plasma Physics and Controlled Fusion Plasma Physics and Controlled Fusion Plasma Phys. Control. Fusion Plasma Phys. Control. Fusion 57 (2015) 075011 (7pp) doi:10.1088/0741-3335/57/7/075011 57 Enhancement of laser to x-ray conversion 2015 by a double-foil gold target © 2015 IOP Publishing Ltd Z Y Ge1, R Ramis2, X H Yang1, T P Yu1, B B Xu1, Y Zhao1, H B Zhuo1, Y Y Ma1, W Yu3 and X J Peng4 PPCF 1 College of Science, National University of Defense Technology, Changsha 410073, People’s Republic of China 075011 2 E.T.S.I. Aeronáuticos, Universidad Politécnica de Madrid, Madrid 28040, Spain 3 Shanghai Institute of Optics and Fine Mechanics, Chinese Academy of Sciences, Shanghai 201800, Z Y Ge et al People’s Republic of China 4 Institute of Applied Physics and Computational Mathematics, Beijing 100088, People’s Republic of China Enhancement of laser to x-ray conversion by a double-foil gold target E-mail: [email protected] and [email protected] Printed in the UK Received 30 January 2015, revised 10 May 2015 Accepted for publication 19 May 2015 Published 18 June 2015 PPCF Abstract A novel double-foil configuration is proposed to improve the laser to x-ray conversion 10.1088/0741-3335/57/7/075011 efficiency from laser irradiating a solid target. One-dimensional radiation hydrodynamic simulations show that the total x-ray conversion efficiency for the double-foil target is as high as 54.7%, which has a 10% improvement compared with the normal target. -
Calibrated Landsat ETM+ Nonthermal-Band Image Mosaics of Afghanistan
In cooperation with U.S. Agency for International Development Calibrated Landsat ETM+ Nonthermal-Band Image Mosaics of Afghanistan By Philip A. Davis Open-File Report 2006-1345 U.S. Department of the Interior U.S. Geological Survey U.S. Department of the Interior DIRK KEMPTHORNE, Secretary U.S. Geological Survey Mark D. Myers, Director U.S. Geological Survey, Reston, Virginia 20192 2006 For product and ordering information: World Wide Web: http://www.usgs.gov/pubprod Telephone: 1-888-ASK-USGS For more information on the USGS—the Federal source for science about the Earth, its natural and living resources, natural hazards, and the environment: World Wide Web: http://www.usgs.gov Telephone: 1-888-ASK-USGS Suggested citation: Davis, P. A., 2006, Calibrated Landsat ETM+ nonthermal-band image mosaics of Afghanistan, U.S. Geological Survey Open-File Report 2006-1345, 18 p. [http://pubs.usgs.gov/of2006/1345/ ]. Any use of trade, product, or firm names is for descriptive purposes only and does not imply endorsement by the U.S. Government. Although this report is in the public domain, permission must be secured from the individual copyright owners to reproduce any copyrighted material contained within this report. 2 Contents Introduction .........................................................................................................................................................................4 Characteristics of Landsat ETM+ Satellite Image Data ..............................................................................................4 -
Band (32.0 Ghz) and X-Band (8.4 Ghz)
The Characterization of a 34-Meter Beam-Waveguide Antenna at ~-band (32.0 GHz) and X-band (8.4 GHz) David D. Morabito Jet Propulsion Laboratory California Institute of Technology Pasadena, California (Revised Decaber 21, 1998) ABSTRACT New antennas for the NASA Deep Space Network (DSN) have been built to replace the aging antennas of older designs for deep space communications. These new antennas incorporate a new dual-shape design as well as a beam waveguide (BWG), which utilize a series of additional secondary mirrors to relocate the focal point into a stationary room below the main reflector. The advantages of using such a design include increased isolation of the feed package from outside environment tal fact ors such as moisture, wind, and temperature changes and ease of access to the equipment for maintenance, troubleshooting and repair purposes. This article reports on the performance of a beam waveguide antenna at X–band and Ka-band microwave frequencies. The ~-band Antenna Performance Experiment (KaAP) antenna efficiency measurements presented in this article were acquired at the Goldstone DSS-13 ,.+ Research and Development (R&D) beam waveguide (BWG) antenna between December 1993 and November 1995. The measured antenna efficiency and ground station figure-of– merit (gain divided by operating system noise temperature) as a function of elevation angle and their uncertainties are presented. Also described are the station configuration, the measurement technique, the modeling used in the analysis processing, and the historical evolution of the DSS-13 ~-band antenna efficiency measurements as progressive improvements and configuration changes were implemented. 1. INTRODUCTION The Beam-WaveGuide (BWG) design feature had been in use for several years for communications satellite terminals where ease of service outweighed the added noise due to the additional mirrors. -
Mid-Infrared Astronomy with the E-ELT: Performance of METIS
Mid-infrared astronomy with the E-ELT: Performance of METIS S. Kendrewa, L. Jolissainta, B. Brandla, R. Lenzenb, E. Pantinc, A. Glassed, J. Blommaerte, L. Venemaf, R. Siebenmorgeng, F. Molsterh aLeiden Observatory, University of Leiden, PO Box 9513, 2300 RA Leiden, Netherlands; b Max Planck Institute for Astronomy, K¨onigstuhl 17, 69117 Heidelberg, Germany; cCEA Saclay, Gif-sur-Yvette, 91191 cedex, France; dUK Astronomy Technology Centre, Royal Observatory of Edinburgh, Blackford Hill, Edingburgh EH9 3HJ, United Kingdom; eKatholieke Universiteit Leuven, Institute of Astronomy, Celestijnenlaan 200D Bus 2401, 3001 Leuven, Belgium; fAstron, Oude Hoogeveensedijk 4, 7991 PD Dwingeloo, Netherlands; gESO, Karl SchwarzschildStrasse 2, 85748 Garching, Germany. hNOVA,PO Box 9513, 2300 RA Leiden, Netherlands. ABSTRACT We present results of performance modelling for METIS, the Mid-infrared European Extremely Large Telescope Imager and Spectrograph. Designed by a consortium of NOVA (Netherlands), UK Astronomy Technology Centre (UK), MPIA Heidelberg (Germany), CEA Saclay (France) and KU Leuven (Belgium), METIS will cover the atmospheric windows in L, M and N-band and will offer imaging, medium-resolution slit spectroscopy (R 1000- 3000) and high-resolution integral field spectroscopy (R 100,000). Our model uses a detailed set of∼ input parameters for site characteristics and atmospheric profiles,∼ optical design, thermal background and the most up-to-date IR detector specifications. We show that METIS will bring an orders-of-magnitude level improvement in sensitivity and resolution over current ground-based IR facilities, bringing mid-IR sensitivities to the micro- Jansky regime. As the only proposed E-ELT instrument to cover this entire spectral region, and the only mid-IR high-resolution integral field unit planned on the ground or in space, METIS will open up a huge discovery space in IR astronomy in the next decade. -
Ion Irradiation of the Murchison Meteorite: Visible to Mid-Infrared Spectroscopic Results
A&A 577, A41 (2015) Astronomy DOI: 10.1051/0004-6361/201425398 & c ESO 2015 Astrophysics Ion irradiation of the Murchison meteorite: Visible to mid-infrared spectroscopic results C. Lantz1,2, R. Brunetto3, M. A. Barucci1, E. Dartois3, J. Duprat4, C. Engrand4, M. Godard4,D.Ledu4, and E. Quirico5 1 Laboratoire d’Études Spatiales et d’Instrumentation en Astrophysique (LESIA) – Observatoire de Paris, CNRS (UMR 8109)/UPMC (Paris 6) / Univ. Paris Diderot (Paris 7), 92195 Meudon Cedex, France e-mail: [email protected] 2 Université Paris Diderot, Sorbonne Paris Cité, 75205 Paris Cedex 13, France 3 Institut d’Astrophysique Spatiale (IAS), CNRS (UMR 8617)/Université Paris-Sud (Paris 11), 91405 Orsay Cedex, France 4 Centre de Sciences Nucléaires et de Sciences de la Matière (CSNSM), IN2P3 – CNRS (UMR 8609)/Université Paris-Sud (Paris 11), 91405 Orsay Cedex, France 5 Institut de Planétologie et d’Astrophysique de Grenoble (IPAG), Université J. Fournier – Grenoble 1/CNRS-INSU (UMR 5274), 38041 Grenoble Cedex 9, France Received 24 November 2014 / Accepted 3 March 2015 ABSTRACT Aims. The goal of this study is to simulate space weathering processes on primitive bodies. We use ion implantation as a simulation of solar wind irradiation, which has been suggested by several authors to be the major component of space weathering on main belt asteroids. The laboratory analogs we irradiate and analyze are carbonaceous chondrites; we started the study with the Allende CV meteorite and in this companion paper we present results on the Murchison CM meteorite. Methods. We performed irradiations on pressed pellets of Murchison with 40 keV He+ and Ar+ ions using fluences up to 3 × 1016 ions/cm2.