Variability of the Structural Coloration in Two Butterfly Species with Different Prezygotic Mating Strategies
Total Page:16
File Type:pdf, Size:1020Kb
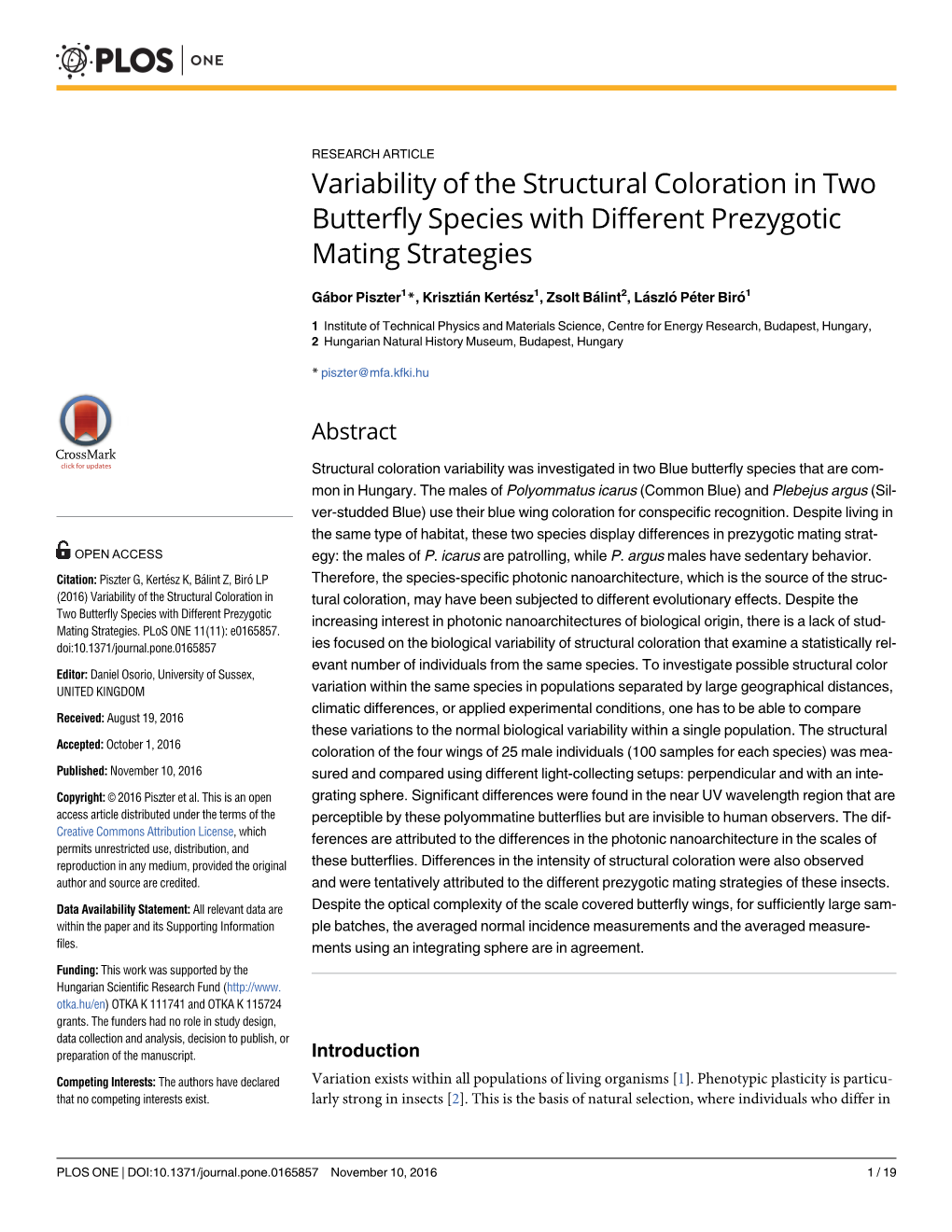
Load more
Recommended publications
-
An IPM Pocket Guide for Weed Identification in Nurseries and Landscapes Produced by Michigan State University Extension
Back to table of contents Back to index 2020 PDF An IPM Pocket Guide for Weed Identification in Nurseries and Landscapes Produced by Michigan State University Extension Contents Index of weeds (clickable) ........................ 2-8 Weed identification ............................... 9-183 Using this scouting guide..........................184 Weed classification ...................................185 Grass and broadleaf characteristics . 186-189 Glossary ........................................... 190-192 Credits and acknowledgments ......... 193-194 Suggested reading ...................................195 1 Back to table of contents Back to index Index By common name Annual bluegrass ...........................................32-33 Annual sowthistle................................................73 Asiatic (common) dayflower ..........................18-19 Barnyardgrass ....................................................27 Bindweed, field ..........................................101-103 Bindweed, hedge .......................................102-103 Birdseye pearlwort ..............................................98 Birdsfoot trefoil...........................................109-110 Bittercress, hairy ............................................83-85 Bittercress, smallflowered...................................85 Black medic ............................................... 112-113 Blackseed plantain ...........................................150 Bluegrass, annual ..........................................32-33 Brambles ...................................................164-165 -
State of New York City's Plants 2018
STATE OF NEW YORK CITY’S PLANTS 2018 Daniel Atha & Brian Boom © 2018 The New York Botanical Garden All rights reserved ISBN 978-0-89327-955-4 Center for Conservation Strategy The New York Botanical Garden 2900 Southern Boulevard Bronx, NY 10458 All photos NYBG staff Citation: Atha, D. and B. Boom. 2018. State of New York City’s Plants 2018. Center for Conservation Strategy. The New York Botanical Garden, Bronx, NY. 132 pp. STATE OF NEW YORK CITY’S PLANTS 2018 4 EXECUTIVE SUMMARY 6 INTRODUCTION 10 DOCUMENTING THE CITY’S PLANTS 10 The Flora of New York City 11 Rare Species 14 Focus on Specific Area 16 Botanical Spectacle: Summer Snow 18 CITIZEN SCIENCE 20 THREATS TO THE CITY’S PLANTS 24 NEW YORK STATE PROHIBITED AND REGULATED INVASIVE SPECIES FOUND IN NEW YORK CITY 26 LOOKING AHEAD 27 CONTRIBUTORS AND ACKNOWLEGMENTS 30 LITERATURE CITED 31 APPENDIX Checklist of the Spontaneous Vascular Plants of New York City 32 Ferns and Fern Allies 35 Gymnosperms 36 Nymphaeales and Magnoliids 37 Monocots 67 Dicots 3 EXECUTIVE SUMMARY This report, State of New York City’s Plants 2018, is the first rankings of rare, threatened, endangered, and extinct species of what is envisioned by the Center for Conservation Strategy known from New York City, and based on this compilation of The New York Botanical Garden as annual updates thirteen percent of the City’s flora is imperiled or extinct in New summarizing the status of the spontaneous plant species of the York City. five boroughs of New York City. This year’s report deals with the City’s vascular plants (ferns and fern allies, gymnosperms, We have begun the process of assessing conservation status and flowering plants), but in the future it is planned to phase in at the local level for all species. -
Dragonflies and Damselflies in Your Garden
Natural England works for people, places and nature to conserve and enhance biodiversity, landscapes and wildlife in rural, urban, coastal and marine areas. Dragonflies and www.naturalengland.org.uk © Natural England 2007 damselflies in your garden ISBN 978-1-84754-015-7 Catalogue code NE21 Written by Caroline Daguet Designed by RR Donnelley Front cover photograph: A male southern hawker dragonfly. This species is the one most commonly seen in gardens. Steve Cham. www.naturalengland.org.uk Dragonflies and damselflies in your garden Dragonflies and damselflies are Modern dragonflies are tiny by amazing insects. They have a long comparison, but are still large and history and modern species are almost spectacular enough to capture the identical to ancestors that flew over attention of anyone walking along a prehistoric forests some 300 million river bank or enjoying a sunny years ago. Some of these ancient afternoon by the garden pond. dragonflies were giants, with This booklet will tell you about the wingspans of up to 70 cm. biology and life-cycles of dragonflies and damselflies, help you to identify some common species, and tell you how you can encourage these insects to visit your garden. Male common blue damselfly. Most damselflies hold their wings against their bodies when at rest. BDS Dragonflies and damselflies belong to Dragonflies the insect order known as Odonata, Dragonflies are usually larger than meaning ‘toothed jaws’. They are often damselflies. They are stronger fliers and referred to collectively as ‘dragonflies’, can often be found well away from but dragonflies and damselflies are two water. When at rest, they hold their distinct groups. -
2017, Jones Road, Near Blackhawk, RAIN (Photo: Michael Dawber)
Edited and Compiled by Rick Cavasin and Jessica E. Linton Toronto Entomologists’ Association Occasional Publication # 48-2018 European Skippers mudpuddling, July 6, 2017, Jones Road, near Blackhawk, RAIN (Photo: Michael Dawber) Dusted Skipper, April 20, 2017, Ipperwash Beach, LAMB American Snout, August 6, 2017, (Photo: Bob Yukich) Dunes Beach, PRIN (Photo: David Kaposi) ISBN: 978-0-921631-53-7 Ontario Lepidoptera 2017 Edited and Compiled by Rick Cavasin and Jessica E. Linton April 2018 Published by the Toronto Entomologists’ Association Toronto, Ontario Production by Jessica Linton TORONTO ENTOMOLOGISTS’ ASSOCIATION Board of Directors: (TEA) Antonia Guidotti: R.O.M. Representative Programs Coordinator The TEA is a non-profit educational and scientific Carolyn King: O.N. Representative organization formed to promote interest in insects, to Publicity Coordinator encourage cooperation among amateur and professional Steve LaForest: Field Trips Coordinator entomologists, to educate and inform non-entomologists about insects, entomology and related fields, to aid in the ONTARIO LEPIDOPTERA preservation of insects and their habitats and to issue Published annually by the Toronto Entomologists’ publications in support of these objectives. Association. The TEA is a registered charity (#1069095-21); all Ontario Lepidoptera 2017 donations are tax creditable. Publication date: April 2018 ISBN: 978-0-921631-53-7 Membership Information: Copyright © TEA for Authors All rights reserved. No part of this publication may be Annual dues: reproduced or used without written permission. Individual-$30 Student-free (Association finances permitting – Information on submitting records, notes and articles to beyond that, a charge of $20 will apply) Ontario Lepidoptera can be obtained by contacting: Family-$35 Jessica E. -
The European Grassland Butterfly Indicator: 1990–2011
EEA Technical report No 11/2013 The European Grassland Butterfly Indicator: 1990–2011 ISSN 1725-2237 EEA Technical report No 11/2013 The European Grassland Butterfly Indicator: 1990–2011 Cover design: EEA Cover photo © Chris van Swaay, Orangetip (Anthocharis cardamines) Layout: EEA/Pia Schmidt Copyright notice © European Environment Agency, 2013 Reproduction is authorised, provided the source is acknowledged, save where otherwise stated. Information about the European Union is available on the Internet. It can be accessed through the Europa server (www.europa.eu). Luxembourg: Publications Office of the European Union, 2013 ISBN 978-92-9213-402-0 ISSN 1725-2237 doi:10.2800/89760 REG.NO. DK-000244 European Environment Agency Kongens Nytorv 6 1050 Copenhagen K Denmark Tel.: +45 33 36 71 00 Fax: +45 33 36 71 99 Web: eea.europa.eu Enquiries: eea.europa.eu/enquiries Contents Contents Acknowledgements .................................................................................................... 6 Summary .................................................................................................................... 7 1 Introduction .......................................................................................................... 9 2 Building the European Grassland Butterfly Indicator ........................................... 12 Fieldwork .............................................................................................................. 12 Grassland butterflies ............................................................................................. -
Butterflies & Flowers of the Kackars
Butterflies and Botany of the Kackars in Turkey Greenwings holiday report 14-22 July 2018 Led by Martin Warren, Yiannis Christofides and Yasemin Konuralp White-bordered Grayling © Alan Woodward Greenwings Wildlife Holidays Tel: 01473 254658 Web: www.greenwings.co.uk Email: [email protected] ©Greenwings 2018 Introduction This was the second year of a tour to see the wonderful array of butterflies and plants in the Kaçkar mountains of north-east Turkey. These rugged mountains rise steeply from Turkey’s Black Sea coast and are an extension of the Caucasus mountains which are considered by the World Wide Fund for Nature to be a global biodiversity hotspot. The Kaçkars are thought to be the richest area for butterflies in this range, a hotspot in a hotspot with over 160 resident species. The valley of the River Çoruh lies at the heart of the Kaçkar and the centre of the trip explored its upper reaches at altitudes of 1,300—2,300m. The area consists of steep-sided valleys with dry Mediterranean vegetation, typically with dense woodland and trees in the valley bottoms interspersed with small hay-meadows. In the upper reaches these merge into alpine meadows with wet flushes and few trees. The highest mountain in the range is Kaçkar Dağı with an elevation of 3,937 metres The tour was centred around the two charming little villages of Barhal and Olgunlar, the latter being at the fur- thest end of the valley that you can reach by car. The area is very remote and only accessed by a narrow road that winds its way up the valley providing extraordinary views that change with every turn. -
Colias Ponteni 47 Years of Investigation, Thought and Speculations Over a Butterfly
Insectifera VOLUME 11 • YEAR 2019 2019 YEAR • SPECIAL ISSUE Colias ponteni 47 years of investigation, thought and speculations over a butterfly INSECTIFERA • YEAR 2019 • VOLUME 11 Insectifera December 2019, Volume 11 Special Issue Editor Pavel Bína & Göran Sjöberg Sjöberg, G. 2019. Colias ponteni Wallengren, 1860. 47 years of investigation, thought and speculations over a butterfly. Insectifera, Vol. 11: 3–100. Contents 4 Summary 4 My own reflections 5 The background to the first Swedish scientific sailing round the world, 1851–1853 16 Extreme sex patches – androconia and antennae 20 Colias ponteni in the collection of BMNH. Where do they come from? Who have collected them and where and when? 22 Two new Colias ponténi and a pupa! 24 Hawaii or Port Famine? Which locality is most likely to be an objective assessment? 25 Colias ponteni - a sensitive "primitive species". Is it extinct? 26 Cause of likely extinction 28 IRMS (Isotope Ratio Mass Spectrometer) isotope investigations 29 What more can suggest that Samuel Pontén's butterflies really were taken in Hawaii? 30 Can Port Famine or the surrounding areas be the right place for Colias ponteni? 34 Collection on Oahu 37 Is there more that suggests that Samuel Pontén found his Colias butterflies during this excursion on Oahu near Honolulu? 38 The background to my studies 39 Is there something that argues against Port Famine as a collection site for Colias ponteni? 39 Is it likely that the butterflies exist or may have been on Mt Tarn just south of Port Famine on the Strait of Magellan? 41 -
Pterygota Winged Insects
pterygota Potamanthus luteus has yellowish unspotted Winged Insects wings, and abdomen barely mar·ked. Local. MA YFLIES (JI/IJ()I)!! dijJ!crlllJl ORDER EPHEMEROPTERA A small species, 1000ISmm long, but dis• tinctive in having only 2 wings and 2 tails. A small and distinctive group, with an un• The female> have yellowish f,'ont mar'gins. usual life-cycle. Unique among insects, they Known to fisher'men as 'pond olives'. have 2 adult phases, moulting again after they Habitat Still and slow-flowing waters. attain the winged state. A sub-adult emerges Status and distribution Common and from the aquatic nymph and takes flight, usu• widespread thl'Oughout. ally hiding among vegetation; this phase is Season 5-10. dull-coloured, with opaque wings, and is known to anglers as the 'dun'. Within hours L/JiJel/lerei/a i,~lli/a the dun moults into the sexually mature A medium-sized species, about 20mm long, adult, which has brighter colours, translu• with a reddish-brown body, reddish-tinged cent wings, and longer tails - the 'spinner'. wings, and 3 tails. The hindwings are small Mayflies are recognizable by the wings, but clearly visible. which are held vertically Habitat Around fast-flowing, well-oxy• above the body, always genated, well-vegetated streams. unfolded; the very shore Status and distribution Widespread antennae; and the 2 or 3 and locally frequent in suitable habitat. long tails. The nymphs Season 4-9. always have 3 tails, even if Similar species the adult has 2. Despite £. notata is yellowish-brown not red, with their name, the short-lived dark stripes and dots under the abdomen. -
7-1 1 May 2007
Volume 7 Number 1 1 May 2007 The Taxonomic Report OF THE INTERNATIONAL LEPIDOPTERA SURVEY A Description of a New Subspecies of Lycaena phlaeas (Lycaenidae: Lycaeninae) from Montana, United States, With a Comparative Study of Old and New World Populations Steve Kohler 125 Hillcrest Loop, Missoula, Montana 59803 United States of America Abstract: The Palaearctic, Oriental and Ethiopian Region subspecies of Lycaena phlaeas are briefly discussed. A more detailed account of the North American subspecies is presented, and a new subspecies, L. p. weberi, from the Sweet Grass Hills, Montana is described. The possibility that the eastern United States subspecies hypophlaeas was introduced from the Old World is discussed; however no conclusion can be reached with certainty. The relationship between Old World and New World subspecies of L. phlaeas is discussed. Evidence presented supports the treatment of New World populations as subspecies of L. phlaeas. Additional key words: Polygonaceae, Rumex acetosella, R. acetosa, R. crispus, Oxyria digyna. INTRODUCTION Lycaena phlaeas (Linnaeus, 1761) is a widespread species with subspecies in Europe, North Africa, Arabia, northern Asia, Japan, North America and tropical Africa. The nominate subspecies occurs in northern Europe (Ackery et al., 1995). Shields & Montgomery (1966) mentioned that European texts list Polygonaceae (Rumex and Polygonum) as larval foodplants for L. phlaeas subspecies. Flight period is April to November, in one to four generations, depending on local conditions; over-wintering is in the larval stage (Tuzov, 2000). Bridges (1988) listed 19 subspecies in his catalogue, not including the North American ones. Miller and Brown (1981) listed five subspecies for North America. Ford (1924) attempted to cover the world-wide geographic races of L. -
SPRING WILDFLOWERS of OHIO Field Guide DIVISION of WILDLIFE 2 INTRODUCTION This Booklet Is Produced by the ODNR Division of Wildlife As a Free Publication
SPRING WILDFLOWERS OF OHIO field guide DIVISION OF WILDLIFE 2 INTRODUCTION This booklet is produced by the ODNR Division of Wildlife as a free publication. This booklet is not for resale. Any By Jim McCormac unauthorized reproduction is prohibited. All images within this booklet are copyrighted by the Division of Wild- life and it’s contributing artists and photographers. For additional information, please call 1-800-WILDLIFE. The Ohio Department of Natural Resources (ODNR) has a long history of promoting wildflower conservation and appreciation. ODNR’s landholdings include 21 state forests, 136 state nature preserves, 74 state parks, and 117 wildlife HOW TO USE THIS GUIDE areas. Collectively, these sites total nearly 600,000 acres Bloom Calendar Scientific Name (Scientific Name Pronunciation) Scientific Name and harbor some of the richest wildflower communities in MID MAR - MID APR Definition BLOOM: FEB MAR APR MAY JUN Ohio. In August of 1990, ODNR Division of Natural Areas and Sanguinaria canadensis (San-gwin-ar-ee-ah • can-ah-den-sis) Sanguinaria = blood, or bleeding • canadensis = of Canada Preserves (DNAP), published a wonderful publication entitled Common Name Bloodroot Ohio Wildflowers, with the tagline “Let Them Live in Your Eye Family Name POPPY FAMILY (Papaveraceae). 2 native Ohio species. DESCRIPTION: .CTIGUJQY[ƃQYGTYKVJPWOGTQWUYJKVGRGVCNU Not Die in Your Hand.” This booklet was authored by the GRJGOGTCNRGVCNUQHVGPHCNNKPIYKVJKPCFC[5KPINGNGCHGPYTCRU UVGOCVƃQYGTKPIVKOGGXGPVWCNN[GZRCPFUKPVQCNCTIGTQWPFGFNGCH YKVJNQDGFOCTIKPUCPFFGGRDCUCNUKPWU -
Description of a New Subspecies of Polyommatus Ciloicus De Freina & Witt, 1983: Alamuticus Ssp
Nachr. entomol. Ver. Apollo, N. F. 27 (3): 171–175 (2006) 171 Description of a new subspecies of Polyommatus ciloicus de Freina & Witt, 1983: alamuticus ssp. n. from North Iran (Alburz Mts.) (Lepidoptera: Lycaenidae) Alireza Naderi and Wolfgang ten Hagen Alireza Naderi, National Natural History Museum of Iran, Tehran, Iran; [email protected] Dr. Wolfgang ten Hagen, Frühlingstraße 1, D-63853 Mömlingen, Germany; [email protected] Abstract: In the present paper a new subspecies of Poly subspecies of P. ciloicus has been detected and described ommatus ciloicus de Freina & Witt, 1983 from western from NW Iran, province Azarbayjan-e Gharbi: P. ciloicus part of Central Alburz (Iran) is described and illustrated: azarisorum Weidenhoffer, 2002. alamuticus ssp. n. (male holotype in coll. Pest and Plant Diseases Research Institute [PPDRI], Tehran, Iran). Males We also provide interesting new distributional infor- of the new taxon can be distinguished by broader wings, mation about the presence of the scarce Pseudophilotes deep blue colour with completely black marginal line on bavius (Eversmann, 1832) at the type locality of the new upperside, fine and definitive markings of underside and totally white fringes. Information on ecology and distribu- subspecies. tion is provided. P. ciloicus from Qazvin can be distinguished from both other subspecies and is described as follows: Eine neue Unterart von Polyommatus ciloicus de Freina & Witt, 1983: alamuticus ssp. n. aus Nordiran Polyommatus ciloicus alamuticus ssp. n. (Lepidoptera: Lycaenidae) Holotype ♂: Iran, Qazvin prov., N. Qazvin, 2000 m, 23. v. Zusammenfassung: Eine neue Subspezies von Polyomma 2005, leg. A. R. Naderi, coll. Pest and Plant Diseases Research tus ciloicus de Freina & Witt, 1983 wird aus dem westlichen Institute (PPDRI), Tehran, Iran. -
Butterflies of the Balkans - Bulgaria, Macedonia and Greece Rd Th Sunday 3 - Sunday 17 July 2016 Trip Report by Dr
Butterflies of the Balkans - Bulgaria, Macedonia and Greece rd th Sunday 3 - Sunday 17 July 2016 Trip report by Dr. Mario Langourov Tour leader: Dr. Mario Langourov1 Tour participants: Mr. Kenneth Bailey Mr. Stephen Meredith Mr. Peter Chadd Mr. Adrian Hoskins Mr.Patrick Nash rd th th th th Bulgaria, 3 – 5 , 19 and 13 – 17 July 2016 th th Macedonia, 5 – 9 July th th Greece, 9 – 12 July rd Day 1 Sunday 3 July Bulgaria – Outbound from Sofia; en route to Rila Monastery. Weather: sunny with some clouds, still & warm (~23ºC). Before noon I welcomed three of the participants at the Terminal 1 and together we went to Terminal 2, where the others were. Before we head for our first hotel, located near the Rila Monastery, we stopped not far from Sofia. There, among the fresh greenery, we found mud puddling Lesser Purple Emperor (Pic. 2) and Purple Emperor, and on the surrounding bushes - Map Butterfly, Holly Blue, Speckled Wood. On the road landed and posed Great Banded Grayling. When we swerved off the main road, we decided to stop and that allowed us to expand the list of the day with Scarce Swallowtail Adonis Blue Purple-shot Copper. On the branches of the bushes we saw Sloe Hairstreak and Ilex Hairstreak, along the stream landed Zephyr Blue and Sandy Grizzled Skipper (Pic. 3). Lattice Brown looked for the deep shadows under the overhanging branches, while Large Blue and Checkered Blue prefered the proximity of its food plants. But we are low on time and we have no choice, but to set off to the hotel.