Vrije Universiteit Brussel New Insights Into the Mechanism of Nucleoside
Total Page:16
File Type:pdf, Size:1020Kb
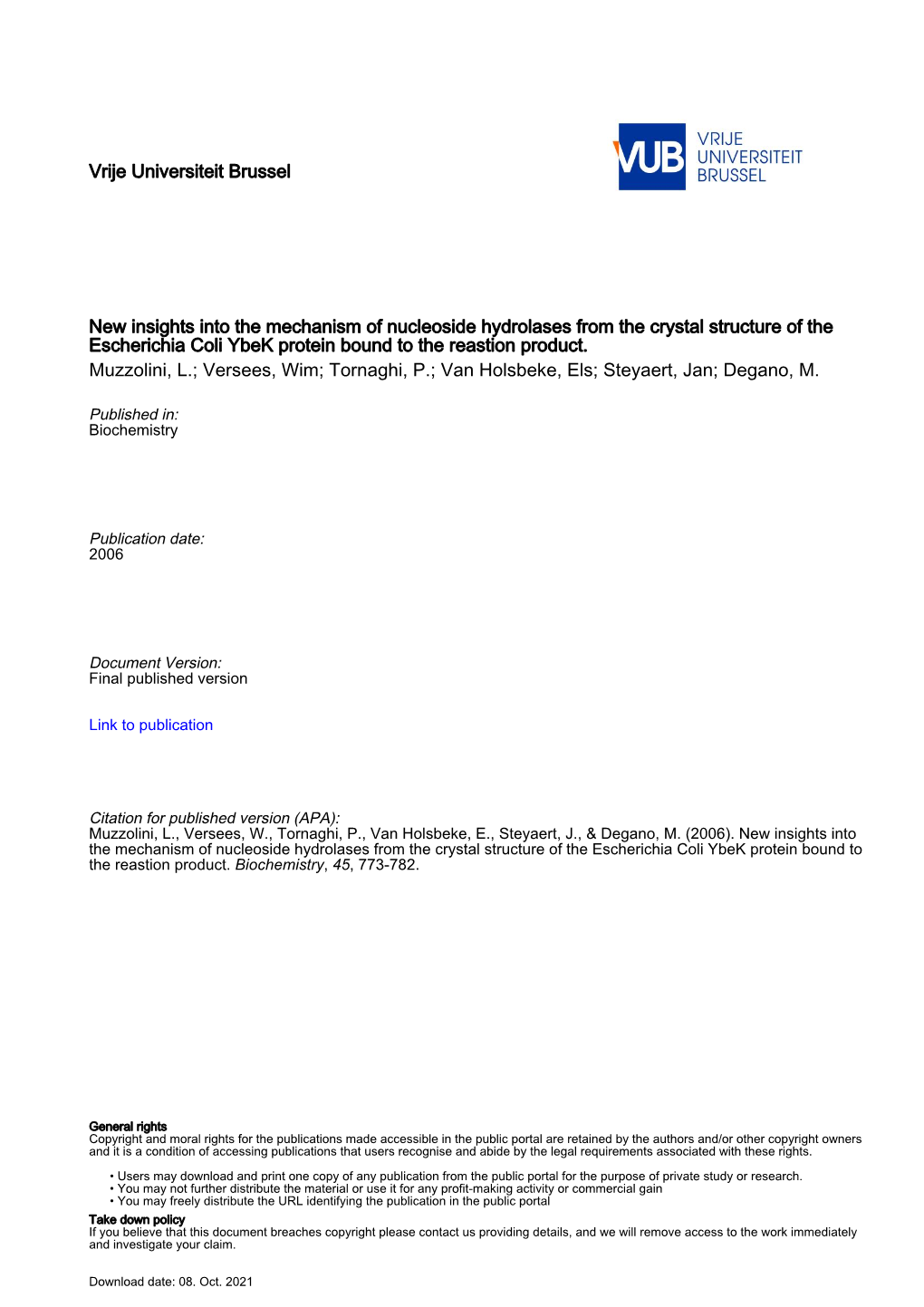
Load more
Recommended publications
-
Downloaded As a Text File, Is Completely Dynamic
BMC Bioinformatics BioMed Central Database Open Access ORENZA: a web resource for studying ORphan ENZyme activities Olivier Lespinet and Bernard Labedan* Address: Institut de Génétique et Microbiologie, CNRS UMR 8621, Université Paris-Sud, Bâtiment 400, 91405 Orsay Cedex, France Email: Olivier Lespinet - [email protected]; Bernard Labedan* - [email protected] * Corresponding author Published: 06 October 2006 Received: 25 July 2006 Accepted: 06 October 2006 BMC Bioinformatics 2006, 7:436 doi:10.1186/1471-2105-7-436 This article is available from: http://www.biomedcentral.com/1471-2105/7/436 © 2006 Lespinet and Labedan; licensee BioMed Central Ltd. This is an Open Access article distributed under the terms of the Creative Commons Attribution License (http://creativecommons.org/licenses/by/2.0), which permits unrestricted use, distribution, and reproduction in any medium, provided the original work is properly cited. Abstract Background: Despite the current availability of several hundreds of thousands of amino acid sequences, more than 36% of the enzyme activities (EC numbers) defined by the Nomenclature Committee of the International Union of Biochemistry and Molecular Biology (NC-IUBMB) are not associated with any amino acid sequence in major public databases. This wide gap separating knowledge of biochemical function and sequence information is found for nearly all classes of enzymes. Thus, there is an urgent need to explore these sequence-less EC numbers, in order to progressively close this gap. Description: We designed ORENZA, a PostgreSQL database of ORphan ENZyme Activities, to collate information about the EC numbers defined by the NC-IUBMB with specific emphasis on orphan enzyme activities. -
The Microbiota-Produced N-Formyl Peptide Fmlf Promotes Obesity-Induced Glucose
Page 1 of 230 Diabetes Title: The microbiota-produced N-formyl peptide fMLF promotes obesity-induced glucose intolerance Joshua Wollam1, Matthew Riopel1, Yong-Jiang Xu1,2, Andrew M. F. Johnson1, Jachelle M. Ofrecio1, Wei Ying1, Dalila El Ouarrat1, Luisa S. Chan3, Andrew W. Han3, Nadir A. Mahmood3, Caitlin N. Ryan3, Yun Sok Lee1, Jeramie D. Watrous1,2, Mahendra D. Chordia4, Dongfeng Pan4, Mohit Jain1,2, Jerrold M. Olefsky1 * Affiliations: 1 Division of Endocrinology & Metabolism, Department of Medicine, University of California, San Diego, La Jolla, California, USA. 2 Department of Pharmacology, University of California, San Diego, La Jolla, California, USA. 3 Second Genome, Inc., South San Francisco, California, USA. 4 Department of Radiology and Medical Imaging, University of Virginia, Charlottesville, VA, USA. * Correspondence to: 858-534-2230, [email protected] Word Count: 4749 Figures: 6 Supplemental Figures: 11 Supplemental Tables: 5 1 Diabetes Publish Ahead of Print, published online April 22, 2019 Diabetes Page 2 of 230 ABSTRACT The composition of the gastrointestinal (GI) microbiota and associated metabolites changes dramatically with diet and the development of obesity. Although many correlations have been described, specific mechanistic links between these changes and glucose homeostasis remain to be defined. Here we show that blood and intestinal levels of the microbiota-produced N-formyl peptide, formyl-methionyl-leucyl-phenylalanine (fMLF), are elevated in high fat diet (HFD)- induced obese mice. Genetic or pharmacological inhibition of the N-formyl peptide receptor Fpr1 leads to increased insulin levels and improved glucose tolerance, dependent upon glucagon- like peptide-1 (GLP-1). Obese Fpr1-knockout (Fpr1-KO) mice also display an altered microbiome, exemplifying the dynamic relationship between host metabolism and microbiota. -
Transcriptome Sequencing and Analysis of the Entomopathogenic
Liu et al. BMC Genomics (2015) 16:106 DOI 10.1186/s12864-015-1269-y RESEARCH ARTICLE Open Access Transcriptome sequencing and analysis of the entomopathogenic fungus Hirsutella sinensis isolated from Ophiocordyceps sinensis Zhi-Qiang Liu1, Shan Lin1, Peter James Baker1, Ling-Fang Wu1, Xiao-Rui Wang1, Hui Wu2, Feng Xu2, Hong-Yan Wang2, Mgavi Elombe Brathwaite3 and Yu-Guo Zheng1* Abstract Background: Ophiocordyceps sinensis, a worm and fungus combined mixture which Hirsutella sinensis is parasitic on the caterpillar body, has been used as a traditional medicine or healthy food in China for thousands of years. H. sinensis is reported as the only correct anamorph of O. sinensis and its main active ingredients are similar to the natural O. sinensis. Results: H. sinensis L0106, asexual strain of O. sinensis, was isolated and identified in this study. Three transcriptomes of H. sinensis at different cultivation periods (growth period 3d, pre-stable period 6d and stable period 9d) were sequenced for the first time by RNA-Seq method, and 25,511 unigenes (3d), 25,214 unigenes (6d) and 16,245 unigenes (9d) were assembled and obtained, respectively. These unigenes of the three samples were further assembled into 20,822 unigenes (All), and 62.3 percent of unigenes (All) could be annotated based on protein databases. Subsequently, the genes and enzymes involved in the biosynthesis of the active ingredients according to the sequencing and annotation results were predicted. Based on the predictions, we further investigated the interaction of different pathway networks and the corresponding enzymes. Furthermore, the differentially expressed genes (DEGs) of H. -
Coupled Nucleoside Phosphorylase Reactions in Escherichia Coli John Lewis Ott Iowa State College
Iowa State University Capstones, Theses and Retrospective Theses and Dissertations Dissertations 1956 Coupled nucleoside phosphorylase reactions in Escherichia coli John Lewis Ott Iowa State College Follow this and additional works at: https://lib.dr.iastate.edu/rtd Part of the Biochemistry Commons, and the Microbiology Commons Recommended Citation Ott, John Lewis, "Coupled nucleoside phosphorylase reactions in Escherichia coli " (1956). Retrospective Theses and Dissertations. 13758. https://lib.dr.iastate.edu/rtd/13758 This Dissertation is brought to you for free and open access by the Iowa State University Capstones, Theses and Dissertations at Iowa State University Digital Repository. It has been accepted for inclusion in Retrospective Theses and Dissertations by an authorized administrator of Iowa State University Digital Repository. For more information, please contact [email protected]. NOTE TO USERS This reproduction is the best copy available. UMI COUPLED NUCLEOSIDE PHOSPHORYLASE REACTIONS IN ESCHERICHIA COLI / by John Lewis Ott A Dissertation Submitted to the Graduate Faculty in Partial Fulfillment of The Requirements for the Degree of DOCTOR OF PHILOSOPHY Major Subject: Physlolgglcal Bacteriology Approved: Signature was redacted for privacy. In Charge of Major Work Signature was redacted for privacy. Head of Major Department Signature was redacted for privacy. Dean of Graduate College Iowa State College 1956 UMI Number: DP12892 INFORMATION TO USERS The quality of this reproduction is dependent upon the quality of the copy submitted. Broken or indistinct print, colored or poor quality illustrations and photographs, print bleed-through, substandard margins, and improper alignment can adversely affect reproduction. In the unlikely event that the author did not send a complete manuscript and there are missing pages, these will be noted. -
Micrtxilnns International 300 N.Zeeb Road Ann Arbor, Ml 48106
INFORMATION TO USERS This reproduction was made from a copy of a document sent to us for microfilming. While the most advanced technology has been used to photograph and reproduce this document, the quality of the reproduction is heavily dependent upon the quality of the material submitted. The following explanation of techniques is provided to help clarify markings or notations which may appear on this reproduction. 1. The sign or “target” for pages apparently lacking from the document photographed is “Missing Page(s)”. If it was possible to obtain the missing page(s) or section, they are spliced into the film along with adjacent pages. This may have necessitated cutting through an image and duplicating adjacent pages to assure complete continuity. 2. When an image on the film is obliterated with a round black mark, it is an indication of either blurred copy because of movement during exposure, duplicate copy, or copyrighted materials that should not have been filmed. For blurred pages, a good image of the page can be found in the adjacent frame. If copyrighted materials were deleted, a target note wül appear listing the pages in the adjacent frame. 3. When a map, drawing or chart, etc., is part of the material being photographed, a definite method of “sectioning” the material has been followed. It is customary to begin filming at the upper left hand comer of a large sheet and to continue from left to right m equal sections with small overlaps. If necessary, sectioning is continued again—beginning below the first row and continuing on until complete. -
12) United States Patent (10
US007635572B2 (12) UnitedO States Patent (10) Patent No.: US 7,635,572 B2 Zhou et al. (45) Date of Patent: Dec. 22, 2009 (54) METHODS FOR CONDUCTING ASSAYS FOR 5,506,121 A 4/1996 Skerra et al. ENZYME ACTIVITY ON PROTEIN 5,510,270 A 4/1996 Fodor et al. MICROARRAYS 5,512,492 A 4/1996 Herron et al. 5,516,635 A 5/1996 Ekins et al. (75) Inventors: Fang X. Zhou, New Haven, CT (US); 5,532,128 A 7/1996 Eggers Barry Schweitzer, Cheshire, CT (US) 5,538,897 A 7/1996 Yates, III et al. s s 5,541,070 A 7/1996 Kauvar (73) Assignee: Life Technologies Corporation, .. S.E. al Carlsbad, CA (US) 5,585,069 A 12/1996 Zanzucchi et al. 5,585,639 A 12/1996 Dorsel et al. (*) Notice: Subject to any disclaimer, the term of this 5,593,838 A 1/1997 Zanzucchi et al. patent is extended or adjusted under 35 5,605,662 A 2f1997 Heller et al. U.S.C. 154(b) by 0 days. 5,620,850 A 4/1997 Bamdad et al. 5,624,711 A 4/1997 Sundberg et al. (21) Appl. No.: 10/865,431 5,627,369 A 5/1997 Vestal et al. 5,629,213 A 5/1997 Kornguth et al. (22) Filed: Jun. 9, 2004 (Continued) (65) Prior Publication Data FOREIGN PATENT DOCUMENTS US 2005/O118665 A1 Jun. 2, 2005 EP 596421 10, 1993 EP 0619321 12/1994 (51) Int. Cl. EP O664452 7, 1995 CI2O 1/50 (2006.01) EP O818467 1, 1998 (52) U.S. -
Study of Nucleoside Degrading Enzyme Activities in Bean, Organic Bean, Okra, Organic Okra, Squash and Organic Squash
STUDY OF NUCLEOSIDE DEGRADING ENZYME ACTIVITIES IN BEAN, ORGANIC BEAN, OKRA, ORGANIC OKRA, SQUASH AND ORGANIC SQUASH by Shafiqa A. Alshaiban A Thesis Submitted in Partial Fulfillment of the Requirements for the Degree of Master of Science in Chemistry Middle Tennessee State University August 2016 Thesis Committee: Dr. Paul C. Kline, Chair Dr. Andrew Burden Dr. Anthony Farone I dedicate this research to my parents, my sisters, and my brothers. I love you all. ii ACKNOWLEDGEMENTS I would like to sincerely thank Dr. Paul Kline for the support and guidance he has offered throughout the entirety of the research and thesis writing process. I also wish to thank my committee members, Dr. Donald A. Burden and Dr. Anthony Farone for their advice and willingness to read my work. I would like to thank all the staff and faculty members for their valuable support. Finally, I would like to express my thanks to my family and my friends for their support and love. Special thanks to my loving parents, my brothers and my sisters for their prayer, concern and kind words over the years. iii ABSTRACT Pyrimidine and purine nucleotide metabolism are essential for development and growth of all organisms. Nucleoside degradation reactions have been found in virtually all organisms. Many enzymes are involved in the degradation and salvage of nucleotides, nucleobases and nucleosides. Deaminases contribute in interconversion of one nucleoside into another by removing amino groups from the base. Nucleoside hydrolase is a glycosidase that catalyzes the cleavage of the N-glycosidic bond in nucleosides to facilitate recycling of nucleobases. -
Purine Metabolism
PURINE METABOLISM CH O-P CH O-P CH O-P CH O-P NH NH NH NH 2 2 2 2 H C 2 H C HC O H O H O O NHCOCH NH 2 2 H2C NH2 2 2 Formyl- CHO CHO CH Glutamine Glutamate OC OC C C H H H H H H Glycine H H or NH H folate NH ADP N H OH H H H H 4 ATP Pi HN = NH ADP ATP AMP O-P-O-P HO H ATP ADP+P Gln H O Glu H N 2 PP H folate 2 ATP Pi 2 OH OH OH OH OH OH OH OH Ribose-P 4 Ribose-P Ribose-P Ribose-P 5-P-ribose 5-P-ribosyl- 5-P-Ribosylamine N1-(5-P-ribosyl)glycinamide N2-formyl-N1-(5-P-ribosyl) 2-(formamido)-N1- 5-amino-1-(P-ribosyl)- 2.7.6.1 pyrophosphate 2.4.2.14 6.3.4.13 2.1.2.2 6.3.5.3 (5-P-ribosyl)acetamidine 6.3.3.1 ADP GDP AMP GTP glycinamide - imidazole (PRPP) GDP ADP ATP ATPGMP -OOCCHCH COO- -OOCCHCH COO- 2 2 O O O CO NH - - - - 2 OOCCHCH COO C HN CO OOCCHCH2COO - C NH 2 C N C NH NH NH OOC 4.1.1.21 N 2 HN H N + NH N C C H2N C 2 C C Aspartate NH3 C CH GDP CH CH Formyl- CH CH Aspartate CH ADP HC C HC C OHC C H4folate C C Pi N Pi N H O N N N ADP C N GTP N 2 N H N H N N H4folate 2 Fumarate 2 P H N H i ATP 2 Ribose-P Ribose-P Ribose-P Ribose-P Ribose-P Ribose-P CLE 4.3.2.2 CY 4.3.2.2 E Adenylosuccinate Inosine- 5-formamido-1-(5-P-ribosyl)- 5-amino-1-(5-P-ribosyl)- 5-amino-1-(5-P-ribosyl-) 5-amino-1-(5-P-ribosyl) ID P 2.1.2.3 T 3.5.4.10 6.3.2.6 O imidazole-4-carboxamide imidazole-4-(N)-[1,2-dicarboxy- imidazole-4-carboxylate E IMP imidazole-4-carboxamide L ethyl]-carboxamide C U N E RNA N I 2.7.7.6 2.7.7.6 R Fumarate TCA U CYCLE P RNA ACIDS RNA RNA n NUCLEIC n n+1 RNAn+1 DNA NH2 NH C 2.7.7.7 2.7.7.7 2 N O C N N C N C CH DNA DNA DNA C N CH HC C n n+1 DNAn+1 -
Green Fluorescent Carbon Dots As Targeting Probes for LED-Dependent Bacterial Killing
Green Fluorescent Carbon Dots as Targeting Probes for LED-Dependent Bacterial Killing Jenny Samphire1,2#, Yuiko Takebayashi2#, Stephen A. Hill1, Nicholas Hill2, Kate J. Heesom3, Philip A. Lewis3, Dominic Alibhai4, Eilis C. Bragginton2, Josephine Dorh5, Neciah Dorh5, James Spencer2*, M. Carmen Galan1* #Equal contribution *[email protected] and [email protected] 1School of Chemistry, Cantock's Close, BS8 1TS, University of Bristol; 2School of Cellular & Molecular Medicine, Biomedical Sciences Building, BS8 1QU, University of Bristol; 3Proteomics Facility, Biomedical Sciences Building, BS8 1TD, University of Bristol; 4Wolfson Bioimaging Facility, Biomedical Sciences Building, BS8 1QU, University of Bristol; 5FluoretiQ Limited, Unit DX, St Philips Central, Albert Road, BS2 0XJ, Bristol, UK Contents: Page number: FCD labelling of different bacterial species S2 Comparison of core-FCDs and FCDs S2 Bacterial growth curves S3 Viable count at different LED irradiation durations S4 2,5-deoxyfructosazine concentration estimation on FCDs S5 Photothermal effect S5 TMT proteomics S6 S1 FCD labelling of different bacterial species Labelling of E. coli, S. aureus, K. pneumoniae and P. aeruginosa with green carbon dots at different concentrations of FCD FCD uptake at different inital FCD concentrations 45 25 µg/mL g/mL) 40 µ 35 50 µg/mL 30 100 µg/mL 25 200 µg/mL 20 15 400 µg/mL 10 5 0 Concentration of FCDs ( FCDs of Concentration E. coli S. aureus P. aeruginosa K. pneumoniae Figure S1. Labelling concentration of FCD with different species of bacteria at five different starting FCD concentrations Comparison of core-FCDs and FCDs Labelling of FCDs without further purification and dialysed FCDs, the majority of 2,5- deoxyfructosazine stripped from the surface. -
All Enzymes in BRENDA™ the Comprehensive Enzyme Information System
All enzymes in BRENDA™ The Comprehensive Enzyme Information System http://www.brenda-enzymes.org/index.php4?page=information/all_enzymes.php4 1.1.1.1 alcohol dehydrogenase 1.1.1.B1 D-arabitol-phosphate dehydrogenase 1.1.1.2 alcohol dehydrogenase (NADP+) 1.1.1.B3 (S)-specific secondary alcohol dehydrogenase 1.1.1.3 homoserine dehydrogenase 1.1.1.B4 (R)-specific secondary alcohol dehydrogenase 1.1.1.4 (R,R)-butanediol dehydrogenase 1.1.1.5 acetoin dehydrogenase 1.1.1.B5 NADP-retinol dehydrogenase 1.1.1.6 glycerol dehydrogenase 1.1.1.7 propanediol-phosphate dehydrogenase 1.1.1.8 glycerol-3-phosphate dehydrogenase (NAD+) 1.1.1.9 D-xylulose reductase 1.1.1.10 L-xylulose reductase 1.1.1.11 D-arabinitol 4-dehydrogenase 1.1.1.12 L-arabinitol 4-dehydrogenase 1.1.1.13 L-arabinitol 2-dehydrogenase 1.1.1.14 L-iditol 2-dehydrogenase 1.1.1.15 D-iditol 2-dehydrogenase 1.1.1.16 galactitol 2-dehydrogenase 1.1.1.17 mannitol-1-phosphate 5-dehydrogenase 1.1.1.18 inositol 2-dehydrogenase 1.1.1.19 glucuronate reductase 1.1.1.20 glucuronolactone reductase 1.1.1.21 aldehyde reductase 1.1.1.22 UDP-glucose 6-dehydrogenase 1.1.1.23 histidinol dehydrogenase 1.1.1.24 quinate dehydrogenase 1.1.1.25 shikimate dehydrogenase 1.1.1.26 glyoxylate reductase 1.1.1.27 L-lactate dehydrogenase 1.1.1.28 D-lactate dehydrogenase 1.1.1.29 glycerate dehydrogenase 1.1.1.30 3-hydroxybutyrate dehydrogenase 1.1.1.31 3-hydroxyisobutyrate dehydrogenase 1.1.1.32 mevaldate reductase 1.1.1.33 mevaldate reductase (NADPH) 1.1.1.34 hydroxymethylglutaryl-CoA reductase (NADPH) 1.1.1.35 3-hydroxyacyl-CoA -
By Lendsey Breanna Thicklin a Thesis Submitt
Adenosine Deaminating/ Hydrolyzing Enzymes from Alaska pea seeds (Pisum sativum) by Lendsey Breanna Thicklin A Thesis Submitted to the Faculty of the College of Graduate Studies in Partial Fulfillment of the Requirements for the Degree of Master of Science in Chemistry Middle Tennessee State University August 2017 Thesis Committee: Dr. Paul C. Kline, Chair Dr. Donald A. Burden Dr. Justin M. Miller I dedicate this thesis research to my ancestors, parents, siblings, mentors, line sisters, and college friends. You all fuel my drive to reach for all of my dreams; no matter how big or small. “Thicklin’s make a difference, we don’t give up” - Dad !ii ACKNOWLEDGEMENTS I am thankful for the financial and academic resources the College of Graduate Studies, College of Basic and Applied Science, and the Department of Chemistry have bestowed to me as a graduate student. Moreover I am grateful for my thesis advisor, Dr. Paul Kline investing in the success and development of this work. I also extend many thanks to my committee members, Dr. Miller and Dr. Burden for their insightful comments, scientific input, and lending reagents and supplies to further develop this work. I am honored and humbled by Dr. Leah Martin’s support as my GTA advisor and mentor. For every pitfall and accomplishment, she has been there to feed me, pray for me, and remind me that there is more greatness to come. Moreover, I thankful for my Crossfit Rampage coach, Korey Akers, and my RamFam community for the physical and mental motivation to push through those “last reps” of grad school! Lastly, I extend my gratitude, hope, and love to my fellow Black female chemists I met at NOBCChE and world-wide. -
NUCLEOSIDE CATABOLIZING ACTIVITIES in SELECTED SEEDS by Nogood Almarwani a Thesis Submitted to the Faculty of the College Of
NUCLEOSIDE CATABOLIZING ACTIVITIES IN SELECTED SEEDS By Nogood Almarwani A Thesis Submitted to the Faculty of the College of Graduate Studies at Middle Tennessee State University in Partial Fulfillment of the Requirements for the Degree of Master of Science in Chemistry Middle Tennessee State University December 2016 Thesis Committee: Dr. Paul C. Kline, Chair Dr. Andrew Burden Dr. Anthony Farone I dedicate this research work to my husband, parents, my sisters, and my brothers. ii ACKNOWLEDGMENTS This research work would not be possible without the support of a great people around me. In the beginning, I would like to thank my loving husband, Fahad Almarwani, for his endless encouragement and support me. I would also like to thank Dr. Paul C. Kline, my major professor. I cannot convey how much I appreciate him for his help and support me. Also, I would like to thank Dr. Anthony Farone and Dr. Andrew Burden as my committee members. I am grateful for their invaluable suggestions and for taking time to read my thesis. In addition, I would like to thank all the staff and faculty of the Department of Chemistry for their help and support throughout my stay at MTSU. Finally, special thanks to my parents, my sisters, my brothers, my little son, Sultan, and my friends. I would not have an opportunity to achieve this dream without their support and love. iii ABSTRACT The efficacy of pyrimidine and purine nucleotide metabolism plays a critical role in the progression of biological systems. The process of nucleotide degradation exists in all organisms. The nucleoside degradation and salvage of nucleotides, nucleosides, and nucleobases require several enzymes including deaminases and nucleosidases.