Observations of Shear Flows in High-Energy-Density Plasmas
Total Page:16
File Type:pdf, Size:1020Kb
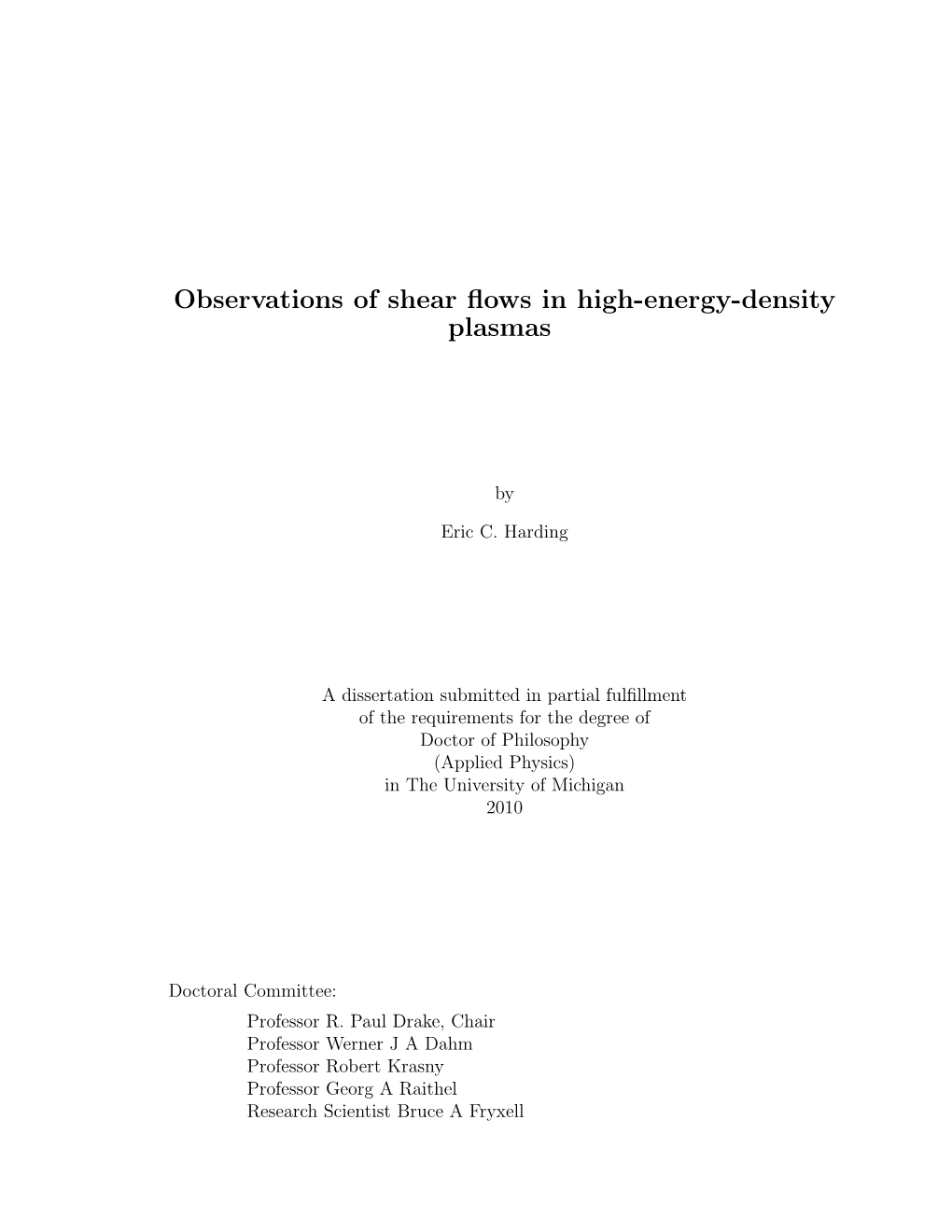
Load more
Recommended publications
-
The Next Generation of Fusion Energy Research
THE NEXT GENERATION OF FUSION ENERGY RESEARCH HEARING BEFORE THE SUBCOMMITTEE ON ENERGY AND ENVIRONMENT COMMITTEE ON SCIENCE AND TECHNOLOGY HOUSE OF REPRESENTATIVES ONE HUNDRED ELEVENTH CONGRESS FIRST SESSION OCTOBER 29, 2009 Serial No. 111–61 Printed for the use of the Committee on Science and Technology ( Available via the World Wide Web: http://www.science.house.gov U.S. GOVERNMENT PRINTING OFFICE 52–894PDF WASHINGTON : 2010 For sale by the Superintendent of Documents, U.S. Government Printing Office Internet: bookstore.gpo.gov Phone: toll free (866) 512–1800; DC area (202) 512–1800 Fax: (202) 512–2104 Mail: Stop IDCC, Washington, DC 20402–0001 COMMITTEE ON SCIENCE AND TECHNOLOGY HON. BART GORDON, Tennessee, Chair JERRY F. COSTELLO, Illinois RALPH M. HALL, Texas EDDIE BERNICE JOHNSON, Texas F. JAMES SENSENBRENNER JR., LYNN C. WOOLSEY, California Wisconsin DAVID WU, Oregon LAMAR S. SMITH, Texas BRIAN BAIRD, Washington DANA ROHRABACHER, California BRAD MILLER, North Carolina ROSCOE G. BARTLETT, Maryland DANIEL LIPINSKI, Illinois VERNON J. EHLERS, Michigan GABRIELLE GIFFORDS, Arizona FRANK D. LUCAS, Oklahoma DONNA F. EDWARDS, Maryland JUDY BIGGERT, Illinois MARCIA L. FUDGE, Ohio W. TODD AKIN, Missouri BEN R. LUJA´ N, New Mexico RANDY NEUGEBAUER, Texas PAUL D. TONKO, New York BOB INGLIS, South Carolina PARKER GRIFFITH, Alabama MICHAEL T. MCCAUL, Texas STEVEN R. ROTHMAN, New Jersey MARIO DIAZ-BALART, Florida JIM MATHESON, Utah BRIAN P. BILBRAY, California LINCOLN DAVIS, Tennessee ADRIAN SMITH, Nebraska BEN CHANDLER, Kentucky PAUL C. BROUN, Georgia RUSS CARNAHAN, Missouri PETE OLSON, Texas BARON P. HILL, Indiana HARRY E. MITCHELL, Arizona CHARLES A. WILSON, Ohio KATHLEEN DAHLKEMPER, Pennsylvania ALAN GRAYSON, Florida SUZANNE M. -
A Fusion Test Facility for Inertial Fusion
A Fusion Test Facility for Inertial Fusion The Fusion Test Facility would be part of an integrated IFE program where the essential elements are developed and implemented as systems in progressively more capable IFE oriented facilities. Fusion Test Facility (FTF) Direct laser drive Sub-megaJoule laser energy Goal of ~150 MW fusion power High flux neutron source Development path to a power plant Presented by Stephen Obenschain U.S. Naval Research Laboratory September 27 2006 Fusion Power Associates Meeting Introduction The scientific underpinnings for ICF has and is being developed via large single shot facilities. The IFE application, while greatly benefiting from this effort, requires a different and broader perspective. • Development of sufficiently efficient and durable high repetition rate drivers. • High gain target designs consistent with the energy application. • Development of economical mass production techniques for targets. • Precision target injection, tracking & laser beam steering. • Reaction chamber design and materials for a harsh environment. • Operating procedures (synchronizing a complex high duty cycle operation) • Overall economics, development time and costs. Individual IFE elements have conflicts in their optimization, and have to be developed in concert with their own purpose-built facilities. HAPL= $25M/yr High Average Power Laser program administered by NNSA See presentations by John Sethian and John Caird this afternoon. Typical GW (electrical )designs have >3 MJ laser drivers @ 5-10 Hz Target Factory Electricity -
Part 1: April 24, 2007 Presentations
UCRL-MI-231268 IFE Science and Technology Strategic Planning Workshop - Part 1: April 24, 2007 Presentations To select an individual presentation, click the table of contents entry on the next page or click the title on the agenda for Day 1 (using the Hand Tool icon). To save only a portion of this document, go to File/Print, select Adobe PDF as your printer, specify the desired range of pages, and save to a new file name. This document was prepared as an account of work sponsored by an agency of the United States Government. Neither the United States Government nor the University of California nor any of their employees, makes any warranty, express or implied, or assumes any legal liability or responsibility for the accuracy, completeness, or usefulness of any information, apparatus, product, or process disclosed, or represents that its use would not infringe privately owned rights. Reference herein to any specific commercial product, process, or service by trade name, trademark, manufacturer, or otherwise, does not necessarily constitute or imply its endorsement, recommendation, or favoring by the United States Government or the University of California. The views and opinions of authors expressed herein do not necessarily state or reflect those of the United States Government or the University of California, and shall not be used for advertising or product endorsement purposes. Portions of this work performed under the auspices of the U. S. Department of Energy by University of California Lawrence Livermore National Laboratory under Contract W-7405-Eng-48. Part 1 Contents Agenda ........................................................................................................................................ 3 Presentations 1. Welcome and Perspectives, Ed Synakowski, LLNL............................................................ -
NRL Nike Laser Focuses on Nuclear Fusion 20 March 2013
NRL Nike Laser focuses on nuclear fusion 20 March 2013 concept, numerous laser beams are used to implode and compress a pea-sized pellet of deuterium-tritium (D-T) to extreme density and temperature, causing the atoms to fuse, resulting in the release of excess energy. In an ICF implosion, a progressively diminishing portion of the beams will engage the shrinking pellet if the focal spot diameter of the laser remains unchanged. For optimal coupling, it becomes desirable to decrease the laser focal spot size to match the reduction in the pellet's diameter, minimizing wasted energy. This is the Nike Laser -- focal zooming. Credit: U.S. "Matching the focal spot size to the pellet Naval Research Laboratory throughout the implosion process maximizes the on- target laser energy," Kehne said. "This experiment validates the engineering of focal zooming in KrF lasers to track the size of an imploding pellet in Researchers at the U.S. Naval Research inertial confinement fusion." Laboratory have successfully demonstrated pulse tailoring, producing a time varying focal spot size With single-step focal zooming implemented, the known as 'focal zooming' on the world's largest Nike laser provides independent control of pulse operating krypton fluoride (KrF) gas laser. shape, time of arrival, and focal diameter allowing greater flexibility in the profiles and pulse shapes The Nike laser is a two to three kilojoule (kJ) KrF that can be produced. The flexibility in pulse system that incorporates beam smoothing by shaping provides promising uses in both future induced spatial incoherence (ISI) to achieve one experiments and laser diagnosis. -
Evaluation of Wavelength Detuning to Mitigate Cross-Beam Energy Transfer Using the Nike Laser
Evaluation of Wavelength Detuning to Mitigate Cross-Beam Energy Transfer Using the Nike Laser Backlighter beams dm: !3 Å KrF 10 J in 400 ps (FWHM*) Drive beams dm: !3 Å (KrF) Target 1.8 kJ in 4 ns 200-nm outer diameter Nike target chamber 57th Annual Meeting of the American Physical Society P. W. McKenty Division of Plasma Physics University of Rochester Savannah, GA Laboratory for Laser Energetics 16–20 November 2015 1 Summary The Nike laser can be employed to examine the effects of laser wavelength detuning to mitigate cross-beam energy transfer (CBET) • Wavelength detuning is predicted to shift the CBET interaction region within the corona, affecting the gains/losses because of CBET • The Nike platform is well suited for these studies, providing a well- diagnosed system over a range of detunings (Dm ~6 Å KrF) • Initial experiments have commenced on Nike, measuring energy dependence of wavelength detuning TC12419 2 Collaborators J. A. Marozas University of Rochester Laboratory for Laser Energetics J. Weaver, S. P. Obenschain, and A. J. Schmitt Naval Research Laboratory 3 Successful wavelength detuning shifts the resonance location sufficiently to mitigate CBET When probe rays are blue-shifted, the resonance shifts to a higher Mach Pump beam number where intersecting probe rays are negligible When probe rays are red-shifted, the resonance shifts to a lower Mach number where probe rays are blocked CBET causes probe rays and/or have negligible intensity Target to extract energy from rc high-intensity pump rays Parabolic locus of turning points Probe beam TC11766e 4 The NIKE experiments will evaluate the disposition of the scattered light at two specific locations OMEGA Nike CBET Target Target Scattered-light disposition TC12420 D. -
IFE/P6-07 Advantages of Krf Lasers for Inertial Confinement Fusion
1 IFE/P6-07 Advantages of KrF Lasers for Inertial Confinement Fusion Energy J. L. Weaver 1), S. P. Obenschain 1), J. D. Sethian 1), A. J. Schmitt 1), V. Serlin 1), R. H. Lehmberg 2), M. Karasik 1), J. Oh 2), J. W. Bates 1), Y. Aglitskiy 3), D. Kehne 1), M. Wolford 1), F. Hegeler 4), M. Myers 1), L. Phillips 5), D. Fyfe 5), D. Colombant 1), E. Mclean 2), W. Manheimer 2), J. Seely 6), U. Feldman 7), M. Klapisch 7), A. L. Velikovich 1), J. Giuliani 1), L. Y Chan 1), S. Zalesak 8), C. Manka 2) 1) Plasma Physics Division, U. S. Naval Research Laboratory, Washington DC, USA 2) Research Support Instruments Inc., Lanham, MD, USA 3) Science Applications International Corporation, Mclean, VA, USA 4) Commonwealth Technology Inc., Alexandria, VA, USA 5) Lab. for Computational Sciences, NRL, Washington, DC, USA 6) Space Science Division, NRL, Washington, DC, USA 7) ARTEP Inc., Ellicot City, MD, USA 8) Berkeley Research Associates Inc., Beltsville, MD, USA E-mail contact of first author: [email protected] Abstract. Advanced concepts for direct drive inertial confinement fusion (ICF) have emerged that may lead to sufficient gain for the energy application (g>140) at laser driver energies as low as 1 megajoule. For example, recent “shock ignition” designs compress low aspect ratio pellets then apply a final high intensity spike pulse (1016 W/cm2) to ignite the fuel via a converging shock wave. These analyses were based on an excimer laser with a Krypton-Fluoride lasing (KrF) medium. KrF systems are particularly well suited to these new ideas as they operate in the deep ultraviolet ( =248 nm), provide highly uniform illumination, possess large bandwidth (1-3 THz), and can easily exploit beam zooming to improve laser-target coupling for the final spike pulse. -
Krf Laser Development A
L+ (j@ fw” 2675-V* / 03 Los Alamos National bboratoq is operated by the University of CaI~ornia for the United States Department of Energy under contract W-7405 -ENG-36 Lowwmm Acknowledgements Technical Review: David C. Cartwnght Joseph F. Figuena Thomas E. McDonald Publication Support: Pa[ncia A. Hinnebusch, C/tiefEdi[or Susrut H. Kinzer, Edi(ou Florence M. Butler, Reference Librarian Lucy P. Maestas, Production A4anagcmen( Mildred E. Valdez, Special Assistant Elizabeth Courtney, Word Processing, Graphics Susan L. Carlson, Graphic Design Support AnnMarie Dyson, llluwations Donna L. Duran, Illustration Support Wendy M. Burditt, Word ProcessinglComposition Joyce A. Martinez, Word ProccssinglComposition Jody Shepard, Assistant Dorothy Hatch, Word Processing Printing Coordination Guadalupe D. Archuleta This document was produced on a Macintosh IITM.LaserWriter Plusw, Varityper VT600Pm, and CanonTM Scanner using Microsoft Wordw v 3.01 and 4.0, Expressionist,w and Adobe Illustratorm. The work reported here was supported by the Office of Inertial Confinement Fusion, Department of Energy — Defense Programs. Front Cover Interior of A URORA target chamber looking directly through the center of the target chamber ton’ard the lens cone. The array of ~’bite circles containing rectangular red stripes in the center of this photograph is a portion of the lens pla(e, some three meters behind the target chamber n’hichfocuses the 48 laser beatns onto the target. The small yellon’ sphere in the center of the chamber is an optical reference target used for optical aiignntent of the laser beants. The ycllom tube entering the chumberfiom (he upper left is part of the x-ray diagnostics system. -
Review of the Department of Energy's Inertial Confinement Fusion
Review of the Department of Energy’s Inertial Confinement Fusion Program Final Report Second Review of the Department of Energy’s Inertial Confinement Fusion Program Final Report Committee for a Second Review of the Department of Energy’s Inertial Confinement Fusion Program Commission on Physical Sciences, Mathematics, and Applications National Research Council National Academy of Sciences 2101 Constitution Avenue Washington, D.C. 204 18 Work Performed under Contract DE-AC0 I -89DP20 154 with the Department of Energy NATIONAL ACADEMY PRESS 2101 Constitution Avenue Washington, D.C. 204 18 September 1990 NOTICE: The project that is the subject of this report was approved by the Governing Board of the National Research Council, whose members are drawn from the councils of the National Academy of Sciences, the National Academy of Engineering, and the Institute of Medicine. The members of the committee responsible for the report were chosen for their special competences and with regard for appropriate balance. This report has been reviewed by a group other than the authors according to procedures approved by a Report Review Committee consisting of members of the National Academy of Sciences, the National Academy of Engineering, and the Institute of Medicine. The National Academy of Sciences is a private, nonprofit, self-perpetuating society of distinguished scholars engaged in scientific and engineering research, dedicated to the furtherance of science and technology and to their use for the general welfare. Upon the authority of the charter granted to it by the Congress in 1863, the Academy has a mandate that requires it to advise the federal government on scientific and technical matters. -
Summary of Opportunities in the Fusion Energy Sciencesprogram
Summary of Opportunities in the Fusion Energy Sciences Program Prepared by the Fusion Energy Sciences Advisory Committee For the Office of Science of the U.S. Department of Energy SUMMARY OF OPPORTUNITIES IN THE FUSION ENERGY SCIENCES PROGRAM November 1999 Prepared by the Fusion Energy Sciences Advisory Committee for the Office of Science of the U.S. Department of Energy Fusion Energy Sciences Advisory Committee Panel * Charles C. Baker, University of California, San Diego Stephen O. Dean, Fusion Power Associates † N. Fisch, Princeton Plasma Physics Laboratory Jeffrey P. Freidberg,* Massachusetts Institute of Technology Richard D. Hazeltine,* University of Texas G. Kulcinski, University of Wisconsin John D. Lindl,* Lawrence Livermore National Laboratory Gerald A. Navratil,* Columbia University Cynthia K. Phillips,* Princeton Plasma Physics Laboratory S. C. Prager, University of Wisconsin D. Rej, Los Alamos National Laboratory John Sheffield,* Oak Ridge National Laboratory, Coordinator J. Soures, University of Rochester R. Stambaugh, General Atomics R. D. Benson, ORNL Support J. Perkins, LLNL Support J. A. Schmidt, PPPL Support N. A. Uckan, ORNL Support * Member of FESAC. † Ex-Member of FESAC. ii Fusion Energy Sciences Advisory Committee 1998–1999 Members Dr. Charles C. Baker, University of California, San Diego Dr. Richard J. Briggs, Science Applications International Corporation Dr. Robert W. Conn, University of California, San Diego Professor Jeffrey P. Freidberg, Massachusetts Institute of Technology Dr. Katharine B. Gebbie, National Institute of Standards and Technology Professor Richard D. Hazeltine, University of Texas at Austin Professor Joseph A. Johnson III, Florida A&M University Dr. John D. Lindl, Lawrence Livermore National Laboratory Dr. Gerald A. Navratil, Columbia University Dr. -
HEDP Study Report
High-Energy-Density Physics Study Report A Comprehensive Study of the Role of High-Energy-Density Physics in the Stockpile Stewardship Program April 6, 2001 High-Energy-Density Physics Study Report A Comprehensive Study of the Role of High-Energy-Density Physics in the Stockpile Stewardship Program April 6, 2001 National Nuclear Security Administration U.S. Department of Energy 1000 Independence Avenue, SW Washington, DC 20585 First Printing, April 9, 2001 Second Printing, April 11, 2001 Third Printing, April 24, 2001 Table of Contents TABLE OF CONTENTS . .i T PREFACE . v A EXECUTIVE SUMMARY . vii B 1 STUDY PURPOSE AND METHODOLOGY . 1 1.1 WORKSHOP METHODOLOGY . .3 L 1.2 QUESTIONS POSED TO FOCUS THE WORKSHOP . .5 1.3 HIGH-ENERGY-DENSITY PHYSICS PROGRAM STUDY . .7 E 2 THE STOCKPILE STEWARDSHIP PROGRAM . 9 2.1 THE SCIENTIFIC APPROACH OF THE STOCKPILE STEWARDSHIP PROGRAM. .12 O 2.2 DELIVERABLES AND OUTCOMES OF THE SSP . .15 F 2.2.1 Annual assessment and certification of the stockpile . .15 2.2.2 Recruiting and Retaining Critically Skilled Personnel into the Stockpile Stewardship Program . .16 C 3 OVERVIEW OF THE BASELINE HIGH-ENERGY-DENSITY PHYSICS PROGRAM. 19 O 3.1 MISSION OF THE HIGH-ENERGY-DENSITY PHYSICS PROGRAM . .21 3.2 PROGRAM ELEMENTS AND PARTICIPATION. .21 N 3.3 PROGRAM REQUIREMENTS. .22 3.4 GOALS OF THE HIGH-ENERGY-DENSITY PHYSICS PROGRAM . .23 T 3.4.1 Execute high-energy-density weapons physics experiments required by the SSP for E ensuring the performance and safety of the nuclear stockpile. .24 3.4.2 Demonstrate ignition at NIF by 2010. -
PLASMA PHYSICS and CONTROLLED NUCLEAR FUSION RESEARCH 1994 VOLUME 4 the Following States Are Members of the International Atomic Energy Agency
Plasma Physics m and Controlled Nuclear Fusion Research 1994 FIFTEENTH CONFERENCE PROCEEDINGS, SEVILLE, SPAIN, 26 SEPTEMBER-1 OCTOBER 1994 Vol.4 Conference Summaries N^ ^ • • :v> *\ The cover picture shows an interior view of the Tokamak Fusion Test Reactor vacuum vessel. At the left, on the inner wall of the vessel, is the bumper limiter composed of graphite and graphite composite tiles. Ion cyclotron radiofrequency launchers are seen at the right along the mid-section of the vacuum vessel wall. By courtesy of the Princeton University Plasma Physics Laboratory. PLASMA PHYSICS AND CONTROLLED NUCLEAR FUSION RESEARCH 1994 VOLUME 4 The following States are Members of the International Atomic Energy Agency: AFGHANISTAN ICELAND PERU ALBANIA INDIA PHILIPPINES ALGERIA INDONESIA POLAND ARGENTINA IRAN, PORTUGAL AUSTRALIA ISLAMIC REPUBLIC OF QATAR ARMENIA IRAQ ROMANIA AUSTRIA IRELAND RUSSIAN FEDERATION BANGLADESH ISRAEL SAUDI ARABIA BELARUS ITALY SENEGAL BELGIUM JAMAICA SIERRA LEONE BOLIVIA JAPAN SINGAPORE BRAZIL JORDAN SLOVAKIA BULGARIA KAZAKHSTAN SLOVENIA CAMBODIA KENYA SOUTH AFRICA CAMEROON KOREA, REPUBLIC OF SPAIN CANADA KUWAIT SRI LANKA CHILE LEBANON SUDAN CHINA LIBERIA SWEDEN COLOMBIA LIBYAN ARAB JAMAHIRIYA SWITZERLAND COSTA RICA LIECHTENSTEIN SYRIAN ARAB REPUBLIC COTE DTVOIRE LITHUANIA, REPUBLIC OF THAILAND CROATIA LUXEMBOURG THE FORMER YUGOSLAV CUBA MADAGASCAR REPUBLIC OF MACEDONIA CYPRUS MALAYSIA TUNISIA CZECH REPUBLIC MALI TURKEY DENMARK MARSHALL ISLANDS UGANDA DOMINICAN REPUBLIC MAURITIUS UKRAINE ECUADOR MEXICO UNITED ARAB EMIRATES EGYPT -
48Th Anomalous Absorption Conference July 8Th-13Th, 2018 Bar
48th Anomalous Absorption Conference July 8th-13th, 2018 Bar Harbor, Maine Organizing Committee Conference Support J. Bates, M. Karasik, J. Weaver Jenifer Schimmenti U. S. Naval Research Laboratory Strategic Analysis, Inc. Washington, DC Arlington, VA Sunday July 8th 6:00 - 10:00 PM Registration 8:00 - 10:00 PM Welcome Reception Monday July 9th Welcome/Opening Remarks 8:30 - 8:50 AM Conference Organizing Committee Oral Session Session Chair: Strozzi, LLNL 8:50 - 9:10 AM MO1 Winjum UCLA Influence of magnetic fields on nonlinear electron plasma waves and kinetic stimulated Raman scattering 9:10 - 9:30 AM MO2 Palastro LLE Resonant absorption of a broadband laser pulse 9:30 - 9:50 AM MO3 Wen UCLA Simulations of stimulated Raman scattering for speckled laser with temporal bandwidth 9:50 - 10:10 AM MO4 Yan Univ. Sci. & Laser-plasma instabilities at large-angle oblique laser incidence Tech. China 10:10 - 10:30 AM Coffee Break Oral Session Session Chair: Albright, LANL 10:30 - 10:50 AM MO5 Molvig LANL/MIT Theory of laser driven ablation 10:50 - 11:10 AM MO6 Sauppe LANL Effects of double shell joint morphology and capsule size on implosion performance 11:10 - 11:30 AM MO7 Schmitt LANL 2-Dimensional simulations of the Revolver direct-drive multi-shell ignition concept 11:30 - 11:50 AM MO8 Wan LANL Experimental investigation of hydrodynamic instability inhibition in a material gradient 11:50 - 12:10 PM MO9 Ho LLNL High-yield implosions via radiation trapping and high rR Evening Invited Talk Session Chair: Casanova, CEA 7:00 - 7:40 PM MI1 Michel