The Complete Chloroplast Genome of Catha Edulis:A Comparative
Total Page:16
File Type:pdf, Size:1020Kb
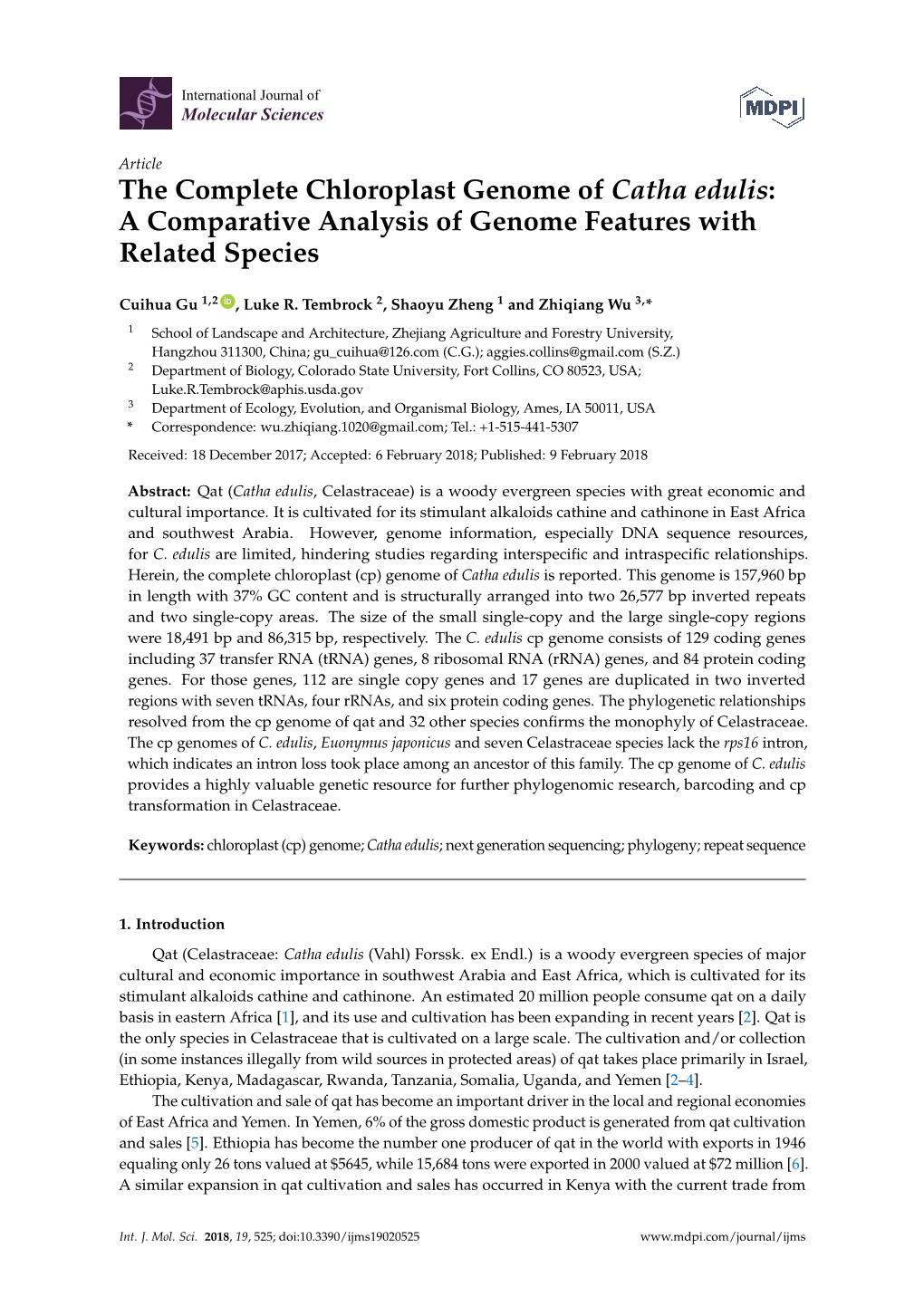
Load more
Recommended publications
-
State of New York City's Plants 2018
STATE OF NEW YORK CITY’S PLANTS 2018 Daniel Atha & Brian Boom © 2018 The New York Botanical Garden All rights reserved ISBN 978-0-89327-955-4 Center for Conservation Strategy The New York Botanical Garden 2900 Southern Boulevard Bronx, NY 10458 All photos NYBG staff Citation: Atha, D. and B. Boom. 2018. State of New York City’s Plants 2018. Center for Conservation Strategy. The New York Botanical Garden, Bronx, NY. 132 pp. STATE OF NEW YORK CITY’S PLANTS 2018 4 EXECUTIVE SUMMARY 6 INTRODUCTION 10 DOCUMENTING THE CITY’S PLANTS 10 The Flora of New York City 11 Rare Species 14 Focus on Specific Area 16 Botanical Spectacle: Summer Snow 18 CITIZEN SCIENCE 20 THREATS TO THE CITY’S PLANTS 24 NEW YORK STATE PROHIBITED AND REGULATED INVASIVE SPECIES FOUND IN NEW YORK CITY 26 LOOKING AHEAD 27 CONTRIBUTORS AND ACKNOWLEGMENTS 30 LITERATURE CITED 31 APPENDIX Checklist of the Spontaneous Vascular Plants of New York City 32 Ferns and Fern Allies 35 Gymnosperms 36 Nymphaeales and Magnoliids 37 Monocots 67 Dicots 3 EXECUTIVE SUMMARY This report, State of New York City’s Plants 2018, is the first rankings of rare, threatened, endangered, and extinct species of what is envisioned by the Center for Conservation Strategy known from New York City, and based on this compilation of The New York Botanical Garden as annual updates thirteen percent of the City’s flora is imperiled or extinct in New summarizing the status of the spontaneous plant species of the York City. five boroughs of New York City. This year’s report deals with the City’s vascular plants (ferns and fern allies, gymnosperms, We have begun the process of assessing conservation status and flowering plants), but in the future it is planned to phase in at the local level for all species. -
Docket 07-Afc-5
DOCKET 07-AFC-5 DATE SEP 24 2008 RECD. SEP 24 2008 Ivanpah Solar Electric Generating System (ISEGS) (07-AFC-5) Supplemental Data Response, Set 1D (Responses to: Biological Resources) Submitted to the California Energy Commission Submitted by Solar Partners I, LLC; Solar Partners II, LLC; Solar Partners IV, LLC; and Solar Partners VIII, LLC September 24, 2008 With Assistance from 2485 Natomas Park Drive Suite 600 Sacramento, CA 95833 Introduction Attached are supplemental responses (Set 1D) by Solar Partners I, LLC; Solar Partners II, LLC; Solar Partners IV, LLC; and Solar Partners VIII, LLC (Applicant) to the California Energy Commission (CEC) Staff’s data requests for the Ivanpah Solar Electric Generating System (Ivanpah SEGS) Project (07-AFC-5). These data requests are the result of the workshop discussion held at Primm, Nevada on June 23, 2008.Within each discipline area, the responses are presented in alphabetical order and are numbered for tracking and reference convenience. New graphics or tables are numbered in reference to the Supplemental Data Request number. For example, if a table were used in response to Data Request AQ-1, it would be numbered Table AQ1-1. The first figure used in response to Data Request AQ-1 would be Figure AQ1-1, and so on. AFC figures or tables that have been revised have “R1” following the original number, indicating revision 1. Additional tables, figures, or documents submitted in response to a supplemental data request (supporting data, stand-alone documents such as plans, folding graphics, etc.) are found at the end of a discipline-specific section and may not be sequentially page-numbered consistently with the remainder of the document, though they may have their own internal page numbering system. -
Millcreek Gardens Sunny Delight Boxleaf Euonymus
Sunny Delight Boxleaf Euonymus* Euonymus japonicus 'Moncliff' Height: 24 inches Spread: 24 inches Sunlight: Hardiness Zone: 5b Other Names: Japanese Spindle Tree Description: Rich glossy dark-green foliage features wide yellow margins; seaside salt tolerance; a great hedge plant with light dense branches that tolerate shearing for a formal appearance, or leave to its natural shape Ornamental Features Sunny Delight Boxleaf Euonymus foliage Sunny Delight Boxleaf Euonymus has attractive dark green foliage Photo courtesy of NetPS Plant Finder edged in yellow. The small glossy oval leaves are highly ornamental and remain dark green throughout the winter. Neither the flowers nor the fruit are ornamentally significant. Landscape Attributes Sunny Delight Boxleaf Euonymus is a dense multi-stemmed evergreen shrub with an upright spreading habit of growth. Its relatively fine texture sets it apart from other landscape plants with less refined foliage. This shrub will require occasional maintenance and upkeep, and can be pruned at anytime. Gardeners should be aware of the following characteristic(s) that may warrant special consideration; - Insects Sunny Delight Boxleaf Euonymus is recommended for the following landscape applications; - Mass Planting - General Garden Use - Topiary Planting & Growing Sunny Delight Boxleaf Euonymus will grow to be about 24 inches tall at maturity, with a spread of 24 inches. It tends to fill out right to the ground and therefore doesn't necessarily require facer plants in front. It grows at a fast rate, and under ideal conditions can be expected to live for 40 years or more. This shrub performs well in both full sun and full shade. It is very adaptable to both dry and moist locations, and should do just fine under typical garden conditions. -
Landscaping Without Harmful Invasive Plants
Landscaping without harmful invasive plants A guide to plants you can use in place of invasive non-natives Supported by: This guide, produced by the wild plant conservation Landscaping charity Plantlife and the Royal Horticultural Society, can help you choose plants that are without less likely to cause problems to the environment harmful should they escape from your planting area. Even the most careful land managers cannot invasive ensure that their plants do not escape and plants establish in nearby habitats (as berries and seeds may be carried away by birds or the wind), so we hope you will fi nd this helpful. A few popular landscaping plants can cause problems for you / your clients and the environment. These are known as invasive non-native plants. Although they comprise a small Under the Wildlife and Countryside minority of the 70,000 or so plant varieties available, the Act, it is an offence to plant, or cause to damage they can do is extensive and may be irreversible. grow in the wild, a number of invasive ©Trevor Renals ©Trevor non-native plants. Government also has powers to ban the sale of invasive Some invasive non-native plants might be plants. At the time of producing this straightforward for you (or your clients) to keep in booklet there were no sales bans, but check if you can tend to the planted area often, but it is worth checking on the websites An unsuspecting sheep fl ounders in a in the wider countryside, where such management river. Invasive Floating Pennywort can below to fi nd the latest legislation is not feasible, these plants can establish and cause cause water to appear as solid ground. -
Olive Family)
Flora of the Carolinas, Virginia, and Georgia, Working Draft of 17 March 2004 -- OLEACEAE OLEACEAE (Olive Family) A family of about 24 genera and 615 species, trees and shrubs, nearly cosmopolitan, but centered in Asia. References: Hardin (1974)=Z. 1 Leaves pinnately compound; fruit a samara; plant a small to large tree . Fraxinus 1 Leaves simple; fruit a drupe or capsule; plant a shrub to small tree. 2 Flowers bright yellow, showy; fruit a many-seeded capsule . Forsythia 2 Flowers white, lilac, or purplish; fruit a drupe or 4-seeded capsule. 3 Leaves cordate or truncate at the base; fruit a 4-seeded capsule; corolla lobes shorter than the tube; flowers lilac or white, in terminal panicles ...............................................................Syringa 3 Leaves cuneate to rounded at the base; fruit a drupe; corolla lobes either shorter or longer than the tube; flowers white or greenish-white, in terminal or lateral panicles or fascicles. 4 Corolla absent; calyx minute or lacking; flowers in axillary fascicles . Forestiera 4 Corolla present (often conspicuous and showy); calyx present; flowers lateral or terminal panicles. 5 Corolla lobes elongate, much longer than the corolla tube . Chionanthus 5 Corolla lobes short, no longer than the corolla tube. 6 Inflorescence a many-flowered terminal panicle; leaves generally ovate, elliptic or lanceolate (widest below or at the middle)....................................................Ligustrum 6 Inflorescence a few-flowered axillary panicle; leaves generally oblanceolate or obovate (widest above the middle)........................................................... Osmanthus Chionanthus Linnaeus (Fringe-tree, Old Man's Beard) A genus of controversial circumscription, either of only 3 species, limited to se. North America and e. Asia, or (if including Linociera) of about 100 species, primarily tropical. -
Plant Shapes Plant Shapes
TheThe AmericanAmerican GARDENERGARDENER® TheThe MagazineMagazine ofof thethe AAmericanmerican HorticulturalHorticultural SocietySociety March / April 2011 designing with Plant Shapes Creation of a Sustainable Rose Garden Daffodils for Every Region Solutions for Landscape Eyesores contents Volume 90, Number 2 . March / April 2011 FEATURES DEPARTMENTS 5 NOTES FROM RIVER FARM 6 MEMBERS’ FORUM 8 NEWS FROM THE AHS River Farm’s Osage orange tree named National Champion, Spring Garden Market in April, National Youth Garden Symposium, ExxonMobil funds summer internship, River Farm part of Historic Garden Week in Virginia, new AHS Affiliate Member program launched. 12 AHS MEMBERS MAKING A DIFFERENCE Honey Barnekoff. 13 AHS CORPORATE MEMBER PROFILE The Espoma Company. 14 AHS NEWS SPECIAL page 18 2011 Great American Gardeners National Award winners and 2011 Book Award winners. DAFFODILS: REGIONAL PROVEN PERFORMERS 18 46 GARDEN SOLUTIONS BY MARY LOU GRIPSHOVER No-sweat tips for great garden soil. Experts from the American Daffodil Society share their recom- mendations for cultivars that will thrive in different regions of 48 HOMEGROWN HARVEST North America. Pleasing peas. 50 GARDENER’S NOTEBOOK A PLANT SHAPE PRIMER BY RAND B. LEE 24 Monarch butterflies make slow recovery, For the design-impaired, here’s how to combine plants with dif- nematodes show promise as fruit tree pest ferent shapes effectively in the garden. biocontrols, Morton Arboretum introduces new sweetspire cultivar, endangered plants lacking in botanic garden collections, OREGON’S PLANT GEEK EXTRAORDINAIRE BY KIM POKORNY 28 Mailorder Gardening Association changes Running a trend-setting nursery, globe-trotting in search of new name, Harold Pellett is 2011 Scott Medal plants, writing horticultural references, and designing gardens recipient. -
Harvard Papers in Botany Volume 22, Number 1 June 2017
Harvard Papers in Botany Volume 22, Number 1 June 2017 A Publication of the Harvard University Herbaria Including The Journal of the Arnold Arboretum Arnold Arboretum Botanical Museum Farlow Herbarium Gray Herbarium Oakes Ames Orchid Herbarium ISSN: 1938-2944 Harvard Papers in Botany Initiated in 1989 Harvard Papers in Botany is a refereed journal that welcomes longer monographic and floristic accounts of plants and fungi, as well as papers concerning economic botany, systematic botany, molecular phylogenetics, the history of botany, and relevant and significant bibliographies, as well as book reviews. Harvard Papers in Botany is open to all who wish to contribute. Instructions for Authors http://huh.harvard.edu/pages/manuscript-preparation Manuscript Submission Manuscripts, including tables and figures, should be submitted via email to [email protected]. The text should be in a major word-processing program in either Microsoft Windows, Apple Macintosh, or a compatible format. Authors should include a submission checklist available at http://huh.harvard.edu/files/herbaria/files/submission-checklist.pdf Availability of Current and Back Issues Harvard Papers in Botany publishes two numbers per year, in June and December. The two numbers of volume 18, 2013 comprised the last issue distributed in printed form. Starting with volume 19, 2014, Harvard Papers in Botany became an electronic serial. It is available by subscription from volume 10, 2005 to the present via BioOne (http://www.bioone. org/). The content of the current issue is freely available at the Harvard University Herbaria & Libraries website (http://huh. harvard.edu/pdf-downloads). The content of back issues is also available from JSTOR (http://www.jstor.org/) volume 1, 1989 through volume 12, 2007 with a five-year moving wall. -
New Plant Records for the Hawaiian Islands 2010–20111
Records of the Hawaii Biological Survey for 2011. Edited by 27 Neal L. Evenhuis & Lucius G. Eldredge. Bishop Museum Occasional Papers 113: 27 –54 (2012) New plant records for the Hawaiian Islands 2010 –2011 1 DANielle FRoHliCH 2 & A lex lAU 2 O‘ahu Early Detection, Bishop Museum, 1525 Bernice Street, Honolulu, Hawai‘i 96817-2704; emails: [email protected]; [email protected] o‘ahu early Detection here documents 26 new naturalized records, 8 new state records, 31 new island records, 1 range extension, and 2 corrections found by us and other indi - viduals and agencies. in addition, several species showing signs of naturalization are men - tioned. A total of 42 plant families are discussed. information regarding the formerly known distribution of flowering plants is based on the Manual of the flowering plants of Hawai‘i (Wagner et al . 1999) and information subse - quently published in the Records of the Hawai ‘i Biological Survey . Voucher specimens are deposited at Bishop Museum’s Herbarium Pacificum (BiSH), Honolulu, Hawai‘i. Acanthaceae Megaskepasma erythroclamys lindau New island record This species, which was previously found naturalizing on o‘ahu, can be distinguished by its 1 –2" long showy burgundy bracts and white, tubular, 2-lipped corollas with 2 fertile stamens (Staples & Herbst 2005). Parker & Parsons (this volume) report this species as naturalized on Hawai‘i island. Material examined . KAUA ‘I: Hā‘ena, in neighborhood makai of highway, near Tunnels Beach, UTM 442390, 2457621. Coastal residential setting; sparingly-branched shrub to 6 ft tall, growing out of a hedge. inflorescence bracts magenta. Species is planted as an ornamental and sparingly natural - ized in the area, 9 Mar 2010, OED 2010030904. -
Alkaloids of Catha Spp. by Courtney Robin Field
ALKALOIDS OF CATHA SPP. BY COURTNEY ROBIN FIELD Submitted in fulfillment of the academic requirements for the degree of MASTERS OF SCIENCE in the DEPARTMENT OF BOTANY FACULTY OF SCIENCE UNIVERSITY OF NATAL PIETERMARITZBURG AUGUST 2001 2 I certifY that, unless specifically indicated to the contrary in the text, this dissertation is the result of my own work. The investigation was carried out in the Department ofBotany, University ofNatal, Pietermaritzburg from January 1996 to August 2001 under the supervision ofDr M. T. Smith. c. R. Field 3 ABSTRACT The levels ofthe psychoactive alkaloids S-(-)-cathinone and its primary metabolite cathine, consisting ofthe diastereomers (+) - norpseudoephedrine and (-)- norephedrine were determined in Catha edulis (Vahl) Forssk. ex Endl., Catha transvaalensis Codd and Catha abbottii Van Wyk & Prins. Alkaloid levels were investigated in C. edulis plants collected from three different localities in South Africa, and one from a Nairobi khat market. The efficiency ofthree different methods for the extraction and isolation ofcathinone and cathine were investigated, viz. an aqueous acid extraction, an organic solvent extraction and an aqueous acid extraction using the commercially available ExtrelutR procedure. The aqueous acid extraction resulted in the rapid loss ofcathinone and yielded variable alkaloid levels in replicate studies. This was also observed when this method was coupled with the ExtrelutR procedure. In contrast, the organic solvent extraction did not result in a loss ofcathinone and provided consistent results over a number of replicates; it also proved to be a simple and rapid method for extracting and isolating cathinone and cathine. A trifluoroacetic acid (TFA) derivatization procedure which has been suggested to produce characteristic diagnostic fragments for gas chromatography / mass spectrometry (GC/MS) identification, was investigated, but failed to produce consistent TFA derivatives ofcathinone and cathine. -
CELASTRACEAE R.Br
FLORA DEL VALLE DE TEHUACÁN-CUICATLÁN Fascículo 76. CELASTRACEAE R.Br. Curtis Clevinger* Jennifer Clevinger* *Plant Resources Center University of Texas at Austin INSTITUTO DE BIOLOGÍA UNIVERSIDAD NACIONAL AUTÓNOMA DE MÉXICO 2010 Primera edición: mayo de 2010 D.R. © 2010 Universidad Nacional Autónoma de México Ciudad Universitaria, Delegación Coyoacán, C.P. 04510, México, Distrito Federal ISBN 968-36-3108-8 Flora del Valle de Tehuacán-Cuicatlán ISBN 978-607-02-1289-5 Fascículo 76 Este fascículo se publica gracias al apoyo económico recibido de la Comisión Nacional para el Conocimiento y Uso de la Biodiversidad. Dirección de los autores: Plant Resources Center University of Texas at Austin 1 University Station F0404 Austin, Texas 78712-0471 USA 1 En la portada: 2 1. Mitrocereus fulviceps (cardón) 2. Beaucarnea purpusii (soyate) 3 4 3. Agave peacockii (maguey fibroso) 4. Agave stricta (gallinita) Dibujo de Elvia Esparza FLORA DEL VALLE DE TEHUACÁN-CUICATLÁN 76: 1-27. 2010 CELASTRACEAE1 R.Br. Curtis Clevinger Jennifer Clevinger Bibliografía. Angiosperm Phylogeny Group. 2009. An update of the Angios- perm Phylogeny Group classification for the orders and families of flowering plants: APG III. Bot. J. Linnean Soc. 161: 105-121. Cronquist, A. 1981. An inte- grated system of classification of flowering plants. Nueva York: Columbia Uni- versity Press. pp. 712-714. Ding Hou, L. 1963. Celastraceae. Flora Malesiana ser. 1, vol 6. págs. Loesener, T. 1942. Celastraceae. In: A. Engler & K. Prantl (eds.). Die Natur. Pflanzenfam. ed. 2. 206: 87-197. Lundell, C.L. 1940. Studies in the American Celastraceae. Bull. Torrey Bot. Club 67: 616-621. Lundell, C.L. -
THE JEPSON GLOBE a Newsletter from the Friends of the Jepson Herbarium
THE JEPSON GLOBE A Newsletter from the Friends of The Jepson Herbarium VOLUME 18 NUMBERS 1 and 2 September 2007 News from the Collections: An update from the Consor- The Charterhouse Herbar- tium of California Herbaria ium by Sula Vanderplank Following my arrival as new Rancho Santa Ana Botanic Garden Administrative Curator in March 2006 (see Globe vol. 17 no. 1) came another The Consortium of California British arrival, the permanent loan of a Herbaria was developed to serve as a Charterhouse School Herbarium from gateway to information from Califor- Godalming in Surrey, England (with the nia vascular plant specimens that are interesting Index Herbariorum acronym housed in herbaria throughout the state. of GOD). This small herbarium (around Currently, ~860,000 specimens from 8,000 specimens) of bound volumes and twelve collections (CHSC, DAV, IRVC, folders dates back to the late eighteenth PGM, RSA-POM, SBBG, SD, SJSU, century and includes specimens from Anna Larsen, 2007 UC-JEPS, UCR, UCSB, and UCSC), all over the British Isles and also col- Welcome! are all searchable through a single lections from Europe, the Middle East, Anna Larsen interface http://ucjeps.berkeley.edu/ South Africa, and east coast United New Education Coordinator consortium/. Four more collections States. The collections are particularly (OBI, HSC, CDA, and CAS-DS) will significant because they document the by Staci Markos be joining in the near future. The par- flora of Greater London during a time In July 2007, Anna Larsen joined ticipating institutions cooperate under of great expansion and many specimens the staff of the Jepson Herbarium as the guidelines of a memorandum of un- represent species no longer found in Coordinator of Public Programs. -
KHAT (Catha Edulis ): the HERB with OFFICIO -LEGAL, SOCIO
The offico-legal, socio-cultural and economic uncertainty of Khat Review Article Khat (Catha edulis): the herb with officio-legal, socio-cultural and economic uncertainty Author: Sikiru Lamina1 ABSTRACT Khat (Catha edulis) is a plant of uncertain and highly controversial status grown in the countries Affiliation: around the Red Sea and on the eastern coast of Africa. The chewing of khat leaves has a deep- 1Physiokinetics and rooted religious and socio-cultural tradition. Khat is considered a cash crop and its cultivation is a Biomedical Technology source of economic value to the societies and nations involved. There have, however, been reports Department, School of negative economic effects on the individuals engaging in the habit of khat chewing. of Health Technology, Federal University of The increasing use of khat worldwide, along with the negative international attention that this Technology, Owerri, has garnered, has led to the present status of uncertainty of the once indigenous practice of khat Nigeria chewing. Scientists, mostly western Europeans, have tended to focus on problems related to khat with little attention to the positive role of khat chewing in society and the world at large. In Correspondence to: addition, no report has directly associated khat with any organised crime, violence or antisocial Sikiru Lamina activity, particularly in countries where khat is legalised. email: This paper reviewed the various areas of uncertainty and controversy relating to khat. Based on [email protected] the findings of the review, further qualitative and quantitative research is required and a positive international approach to khat use at economic, religious and socio-cultural levels is advocated.