The PII Signaling Protein from Red Algae Represents an Evolutionary
Total Page:16
File Type:pdf, Size:1020Kb
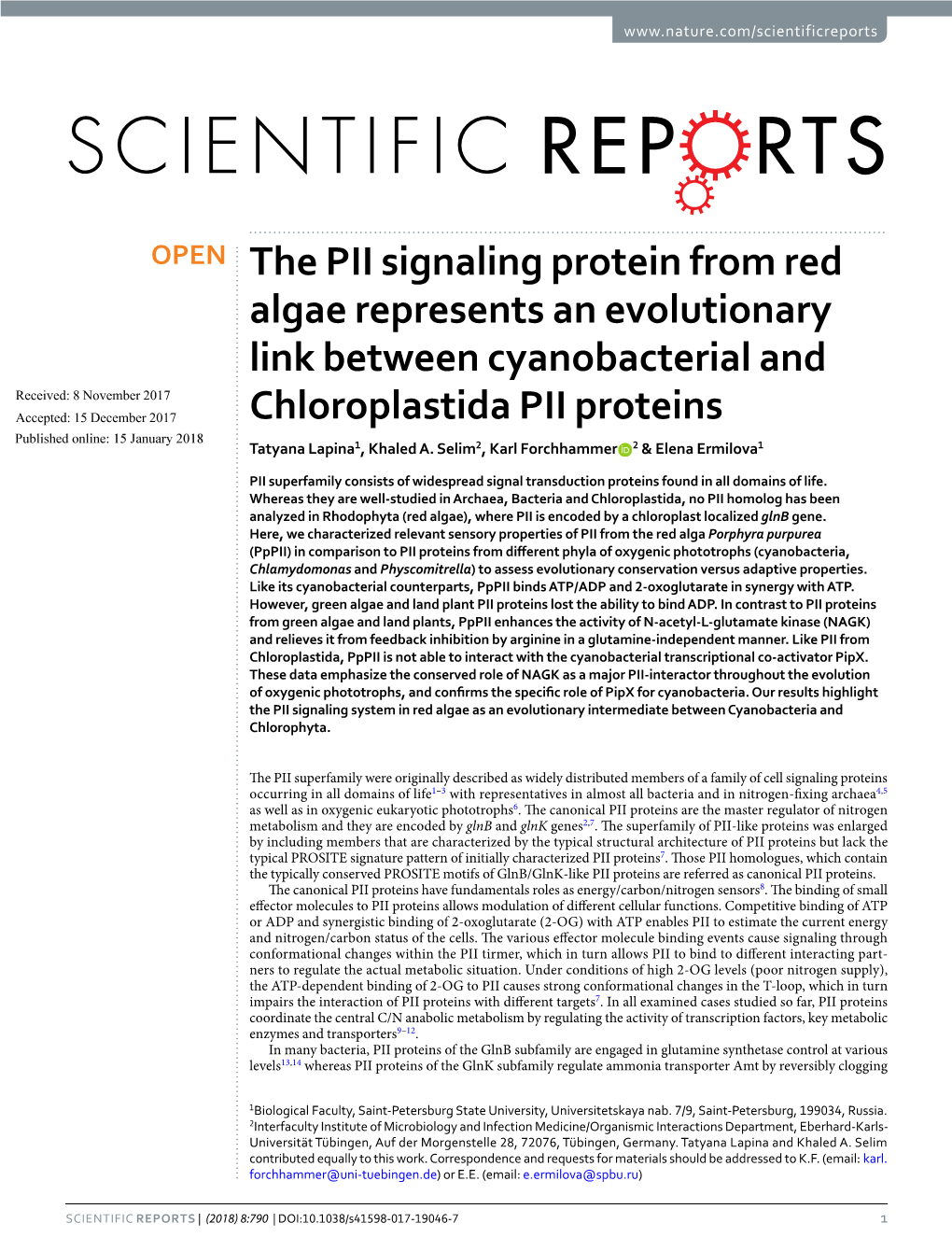
Load more
Recommended publications
-
Genetic Variation Within and Among Asexual Populations of Porphyra Umbilicalis Kützing (Bangiales, Rhodophyta) in the Gulf of Maine, USA Renee L
View metadata, citation and similar papers at core.ac.uk brought to you by CORE provided by UNH Scholars' Repository University of New Hampshire University of New Hampshire Scholars' Repository New Hampshire Agricultural Experiment Station Research Institutes, Centers and Programs 1-13-2016 Genetic variation within and among asexual populations of Porphyra umbilicalis Kützing (Bangiales, Rhodophyta) in the Gulf of Maine, USA Renee L. Eriksen University of New Hampshire, Durham Lindsay A. Green University of New Hampshire, Durham Anita S. Klein University of New Hampshire, Durham, [email protected] Follow this and additional works at: https://scholars.unh.edu/nhaes Recommended Citation Renée L. Eriksen, Lindsay A. Green and Anita S. Klein. Genetic variation within and among asexual populations of Porphyra umbilicalis Kützing (Bangiales, Rhodophyta) in the Gulf of Maine, USA. Botanica Marina 2016; 59(1): 1–12. https://dx.doi.org/ 10.1515/bot-2015-0017 This Article is brought to you for free and open access by the Research Institutes, Centers and Programs at University of New Hampshire Scholars' Repository. It has been accepted for inclusion in New Hampshire Agricultural Experiment Station by an authorized administrator of University of New Hampshire Scholars' Repository. For more information, please contact [email protected]. Botanica Marina 2016; 59(1): 1–12 Renée L. Eriksen*, Lindsay A. Green and Anita S. Klein Genetic variation within and among asexual populations of Porphyra umbilicalis Kützing (Bangiales, Rhodophyta) in the Gulf of Maine, USA DOI 10.1515/bot-2015-0017 Received 16 February, 2015; accepted 8 December, 2015; online first Introduction 13 January, 2016 The marine red alga Porphyra umbilicalis Kützing (Ban- Abstract: The intertidal marine red alga Porphyra umbili- giales, Rhodophyta) is found in the intertidal region on calis reproduces asexually in the Northwest Atlantic. -
Seeding Nets with Neutral Spores Of
Author's personal copy Aquaculture 270 (2007) 77–91 www.elsevier.com/locate/aqua-online Seeding nets with neutral spores of the red alga Porphyra umbilicalis (L.) Kützing for use in integrated multi-trophic aquaculture (IMTA) ⁎ Nicolas Blouin a, , Fei Xiugeng b, Jiang Peng b, Charles Yarish c, Susan H. Brawley a a School of Marine Sciences, 5735 Hitchner Hall, University of Maine, Orono ME 04469, USA b Experimental Marine Biology Laboratory, Institute of Oceanology, Chinese Academy of Sciences, Qingdao 266071, PR China c Department of Ecology and Evolutionary Biology, University of Connecticut, 1 University Place Stamford, CT 06901, USA Received 23 June 2006; received in revised form 5 March 2007; accepted 6 March 2007 Abstract Nets in traditional Porphyra mariculture are seeded with conchospores derived from the conchocelis phase, and spend a nursery period in culture tanks or calm coastal waters until they reach several centimeters in length. Some species of Porphyra can regenerate the foliose phase directly through asexual reproduction, which suggests that the time, infrastructure, and costs associated with conchocelis culture might be avoided by seeding nets with asexual spores. Here, we present work from a short-term mariculture study using nets seeded with asexual spores (neutral spores) of a native Maine species of Porphyra. Porphyra umbilicalis (L.) Kützing was selected for this proof of concept research because of its reproductive biology, abundance across seasons in Maine, and evidence of its promise as a mariculture crop. We studied the maturation, release, and germination of the neutral spores to develop an appropriate seeding protocol for nets, followed by development of a nursery raceway to provide an easily manipulated environment for the seeded nets. -
Seasonal Growth and Phenotypic Variation in Porphyra Linearis (Rhodophyta) Populations on the West Coast of Ireland1
J. Phycol. 43, 90–100 (2007) r 2007 by the Phycological Society of America DOI: 10.1111/j.1529-8817.2006.00300.x SEASONAL GROWTH AND PHENOTYPIC VARIATION IN PORPHYRA LINEARIS (RHODOPHYTA) POPULATIONS ON THE WEST COAST OF IRELAND1 Elena Varela-A´lvarez2 Department of Botany and Martin Ryan Institute, National University of Ireland, Galway, Ireland Dagmar B. Stengel3 and Michael D. Guiry Department of Botany and Martin Ryan Institute, National University of Ireland, Galway, Ireland The phenology and seasonal growth of Porphyra The genus Porphyra was first established by C. Agardh linearis Grev. were investigated in two morphologic- in 1824, and presently there are 267 species names in the ally dissimilar populations from the west coast of AlgaeBase species data base, of which 113 are flagged as Ireland. Thallus size and reproductive status of in- current (Guiry and Guiry 2006). These species generally dividuals were monitored monthly between June occur intertidally or in the shallow subtidal attached to 1997 and June 1998. Both populations exhibited a rocks and other seaweeds. Porphyra (known commonly as similar phenology: gametophyte stages appeared nori in Japan, zicai in China, and purple laver in Great on the shore in October, with spermatangial and zy- Britain) is a major source of food for humans and is the gotosporangial sori appearing the following Febru- most valuable seaweed grown by mariculture in the ary; the gametophyte stage began to degenerate in world today (Hanisak 1998). Several species of Porphyra April and had disappeared completely by June. occur along the North Atlantic coast of Europe, but none However, significant differences in growth and re- of these are currently grown under artificial conditions production in the field and in cultures of plants (Varela-A´lvarez et al. -
Download PDF Version
MarLIN Marine Information Network Information on the species and habitats around the coasts and sea of the British Isles Porphyra purpurea and Ulva spp. on sand-scoured mid or lower eulittoral rock MarLIN – Marine Life Information Network Marine Evidence–based Sensitivity Assessment (MarESA) Review Dr Heidi Tillin & Georgina Budd 2016-03-30 A report from: The Marine Life Information Network, Marine Biological Association of the United Kingdom. Please note. This MarESA report is a dated version of the online review. Please refer to the website for the most up-to-date version [https://www.marlin.ac.uk/habitats/detail/288]. All terms and the MarESA methodology are outlined on the website (https://www.marlin.ac.uk) This review can be cited as: Tillin, H.M. & Budd, G., 2016. [Porphyra purpurea] and [Ulva] spp. on sand-scoured mid or lower eulittoral rock. In Tyler-Walters H. and Hiscock K. (eds) Marine Life Information Network: Biology and Sensitivity Key Information Reviews, [on-line]. Plymouth: Marine Biological Association of the United Kingdom. DOI https://dx.doi.org/10.17031/marlinhab.288.1 The information (TEXT ONLY) provided by the Marine Life Information Network (MarLIN) is licensed under a Creative Commons Attribution-Non-Commercial-Share Alike 2.0 UK: England & Wales License. Note that images and other media featured on this page are each governed by their own terms and conditions and they may or may not be available for reuse. Permissions beyond the scope of this license are available here. Based on a work at www.marlin.ac.uk (page left blank) Date: 2016-03-30 Porphyra purpurea and Ulva spp. -
Pyropia Orbicularis Sp. Nov. (Rhodophyta, Bangiaceae) Based
Pyropia orbicularis sp. nov. (Rhodophyta, Bangiaceae) based on a population previously known as Porphyra columbina from the central coast of Chile Maria-Eliana Ramirez, Loretto Contreras-Porcia, Marie-Laure Guillemin, Juliet Brodie, Catalina Valdivia, María Rosa Flores-Molina, Alejandra Núñez, Cristian Bulboa Contador, Carlos Lovazzano To cite this version: Maria-Eliana Ramirez, Loretto Contreras-Porcia, Marie-Laure Guillemin, Juliet Brodie, Catalina Val- divia, et al.. Pyropia orbicularis sp. nov. (Rhodophyta, Bangiaceae) based on a population previously known as Porphyra columbina from the central coast of Chile. Phytotaxa, Magnolia Press 2014, 158 (2), pp.133-153. hal-01138605 HAL Id: hal-01138605 https://hal.archives-ouvertes.fr/hal-01138605 Submitted on 17 Apr 2015 HAL is a multi-disciplinary open access L’archive ouverte pluridisciplinaire HAL, est archive for the deposit and dissemination of sci- destinée au dépôt et à la diffusion de documents entific research documents, whether they are pub- scientifiques de niveau recherche, publiés ou non, lished or not. The documents may come from émanant des établissements d’enseignement et de teaching and research institutions in France or recherche français ou étrangers, des laboratoires abroad, or from public or private research centers. publics ou privés. 1 Pyropia orbicularis sp. nov. (Rhodophyta, Bangiaceae) based on a 2 population previously known as Porphyra columbina from the central 3 coast of Chile 4 MARÍA ELIANA RAMÍREZ1, LORETTO CONTRERAS-PORCIA2,*, MARIE-LAURE 5 GUILLEMIN3,*, -
Genetic and Morphological Differentiation of Porphyra And
Genetic and morphological differentiation of Porphyra and Pyropia species (Bangiales, Rhodophyta) coexisting in a rocky intertidal in Central Chile Andrés Meynard, Javier Zapata, Nicolás Salas, Claudia Betancourtt, Gabriel Pérez-lara, Francisco Castañeda, María Eliana Ramírez, Cristian Bulboa Contador, Marie-laure Guillemin, Loretto Contreras-porcia To cite this version: Andrés Meynard, Javier Zapata, Nicolás Salas, Claudia Betancourtt, Gabriel Pérez-lara, et al.. Ge- netic and morphological differentiation of Porphyra and Pyropia species (Bangiales, Rhodophyta) coexisting in a rocky intertidal in Central Chile. Journal of Phycology, Wiley, 2019, 55 (2), pp.297- 313. 10.1111/jpy.12829. hal-02147670 HAL Id: hal-02147670 https://hal.sorbonne-universite.fr/hal-02147670 Submitted on 4 Jun 2019 HAL is a multi-disciplinary open access L’archive ouverte pluridisciplinaire HAL, est archive for the deposit and dissemination of sci- destinée au dépôt et à la diffusion de documents entific research documents, whether they are pub- scientifiques de niveau recherche, publiés ou non, lished or not. The documents may come from émanant des établissements d’enseignement et de teaching and research institutions in France or recherche français ou étrangers, des laboratoires abroad, or from public or private research centers. publics ou privés. Journal of Phycology Genetic and morphological differentiation of Porphyra and Pyropia species (Bangiales, Rhodophyta) coexisting in a rocky intertidal in Central Chile Journal: Journal of Phycology Manuscript ID -
Characterization of an Actin Gene Family in Palmaria Palmata and Porphyra Purpurea (Rhodophyta)
Cah. Biol. Mar. (2005) 46 : 311-322 Characterization of an actin gene family in Palmaria palmata and Porphyra purpurea (Rhodophyta) Line LE GALL1, Christophe LELONG, Anne-Marie RUSIG and Pascal FAVREL Laboratoire de Biologie et Biotechnologies Marines, Université de Caen – Basse Normandie UMR 100 Physiologie et Ecophysiologie des Mollusques Marins I.FR.E.MER Esplanade de la Paix, 14032 Caen Cedex, France (1) To whom correspondence should be addressed: CEMAR, Dept of Biology, Mail Service # 45111, University of New Brunswick, Fredericton, NB, E3B 6E1, CANADA Fax: 1 506 453 35 83, Tél: 1 506 453 35 37. E-mail: [email protected] Abstract: The actin gene family was investigated in Palmaria palmata and Porphyra purpurea, two members of the Rhodoplantae. Respectively four and two partial actin gene sequences were isolated from P. purpurea and P. palmata by PCR using actin primers deduced from the conserved regions of many classes of organisms. In P. purpurea, the partial actin gene puract1, puract2 and puract3 were shown to code for proteins whereas puract4 is a pseudogene. This result confirms the presence of several actin genes already suspected in the genus Porphyra. In P. palmata, palmact1 presents a greater identity with actin sequences from other red algae than palmact2, the second gene characterized in this species. Sequencing of the gene palmact1 revealed the existence of an intron in the coding region. To our knowledge this is the first time that two actin encoding genes have been observed in a member of the Florideophyceae. The two genes were both expressed in tetrasporophytic fronds, which raises the question as to their function. -
And Long-Term Freezing on Porphyra Umbilicalis Kützing (Bangiales
© 2014. This manuscript version is made available under the CC-BY-NC-ND 4.0 license http://creativecommons.org/licenses/by-nc-nd/4.0/ doi:10.1016/j.jembe.2014.10.001 1 1 The effects of short- and long-term freezing on Porphyra umbilicalis Kützing (Bangiales, 2 Rhodophyta) blade viability 3 Lindsay A. Green1, 2* and Christopher D. Neefus1 4 1Department of Biological Sciences, University of New Hampshire, 46 College Road, Durham, 5 NH 03824, U.S.A. 6 2Present address: Department of Biological Sciences, University of Rhode Island, 120 Flagg 7 Road, Kingston, RI 02881, U.S.A. 8 *Corresponding author [email protected], +16032644638 9 Abstract 10 Seaweeds inhabiting the upper intertidal zone are subjected to temperature, light, and 11 water stresses and vertical distribution has been linked to environmental tolerance. Previous 12 studies have also attributed successful recovery from freezing stress in intertidal seaweeds to 13 desiccation tolerance. Porphyra umbilicalis Kützing is an aseasonal red alga inhabiting the mid 14 to upper intertidal zone in temperate and subarctic regions of the North Atlantic. It is a member 15 of the economically important group of foliose Bangiales, and has been documented to only 16 reproduce asexually via neutral spores in the Northwest Atlantic. The goal of this study was to 17 assess the effects of freezing on the viability of small blades of P. umbilicalis. Cultured blades of 18 P. umbilicalis (4.8 ±0.22 mg) were air dried to 5% or 30% absolute water content (AWC) and 19 frozen for 1, 3, 6, or 12 months at -80°C or -20°C. -
Insights Into the Red Algae and Eukaryotic Evolution From
Insights into the red algae and eukaryotic evolution PNAS PLUS from the genome of Porphyra umbilicalis (Bangiophyceae, Rhodophyta) Susan H. Brawleya,1, Nicolas A. Blouina,b, Elizabeth Ficko-Bleanc, Glen L. Wheelerd, Martin Lohre, Holly V. Goodsonf, Jerry W. Jenkinsg,h, Crysten E. Blaby-Haasi, Katherine E. Helliwelld,j, Cheong Xin Chank,l, Tara N. Marriagem, Debashish Bhattacharyan, Anita S. Kleino, Yacine Badisp, Juliet Brodieq, Yuanyu Caoo,2, Jonas Collénc, Simon M. Dittamic, Claire M. M. Gachonp, Beverley R. Greenr, Steven J. Karpowiczs, Jay W. Kimt, Ulrich Johan Kudahlj, Senjie Linu, Gurvan Michelc, Maria Mittagv, Bradley J. S. C. Olsonm, Jasmyn L. Pangilinanh, Yi Pengh, Huan Qiun, Shengqiang Shuh, John T. Singerw, Alison G. Smithj, Brittany N. Sprecheru, Volker Wagnerv, Wenfei Wangx, Zhi-Yong Wangy, Juying Yanh, Charles Yarishz, Simone Zäuner-Riekaa, Yunyun Zhuangu,3, Yong Zouv, Erika A. Lindquisth, Jane Grimwoodg,h, Kerrie W. Barryh, Daniel S. Rokhsarh, Jeremy Schmutzg,h, John W. Stillerbb, Arthur R. Grossmany, and Simon E. Prochnikh aSchool of Marine Sciences, University of Maine, Orono, ME 04469; bDepartment of Molecular Biology, University of Wyoming, Laramie, WY 82071; cSorbonne Universités, Université Pierre and Marie Curie Paris 06, CNRS, UMR 8227, Integrative Biology of Marine Models, Station Biologique de Roscoff, CS 90074, 29688 Roscoff, France; dMarine Biological Association of the United Kingdom, Plymouth, PL1 2PB, United Kingdom; eInstitut für Molekulare Physiologie, Pflanzenbiochemie, Johannes Gutenberg-Universität Mainz, -
Health Functionality and Quality Control of Laver (Porphyra, Pyropia): Current Issues and Future Perspectives As an Edible Seaweed
marine drugs Review Health Functionality and Quality Control of Laver (Porphyra, Pyropia): Current Issues and Future Perspectives as an Edible Seaweed Tae Jin Cho and Min Suk Rhee * Department of Biotechnology, College of Life Sciences and Biotechnology, Korea University, 145, Anam-ro, Seongbuk-gu, Seoul 02841, Korea; [email protected] * Correspondence: [email protected]; Tel.: +82-2-3290-3058 Received: 30 November 2019; Accepted: 20 December 2019; Published: 23 December 2019 Abstract: The growing interest in laver as a food product and as a source of substances beneficial to health has led to global consumer demand for laver produced in a limited area of northeastern Asia. Here we review research into the benefits of laver consumption and discuss future perspectives on the improvement of laver product quality. Variation in nutritional/functional values among product types (raw and processed (dried, roasted, or seasoned) laver) makes product-specific nutritional analysis a prerequisite for accurate prediction of health benefits. The effects of drying, roasting, and seasoning on the contents of both beneficial and harmful substances highlight the importance of managing laver processing conditions. Most research into health benefits has focused on substances present at high concentrations in laver (porphyran, Vitamin B12, taurine), with assessment of the expected effects of laver consumption. Mitigation of chemical/microbiological risks and the adoption of novel technologies to exploit under-reported biochemical characteristics of lavers are suggested as key strategies for the further improvement of laver product quality. Comprehensive analysis of the literature regarding laver as a food product and as a source of biomedical compounds highlights the possibilities and challenges for application of laver products. -
Porphyra: Complex Life Histories in a Harsh Environment: P
PORPHYRA: COMPLEX LIFE HISTORIES IN A HARSH ENVIRONMENT: P. UMBILICALIS, AN INTERTIDAL RED ALGA FOR GENOMIC ANALYSIS ELISABETH GANTT1, G. MINE BERG2, DEBASHISH BHATTACHARYA3, NICOLAS A. BLOUIN4, JULIET A. BRODIE5, CHEONG XIN CHAN3, JONAS COLLÉN6, FRANCIS X. CUNNINGHAM JR1, JEFERSON GROSS3, ARTHUR R. GROSSMAN7, STEVEN KARPOWICZ8, YUKIHIRO KITADE9, ANITA S. KLEIN10, IRA A. LEVINE11, SENJIE LIN12, SHAN LU13, MICHAEL LYNCH14, SUBHASH C. MINOCHA10, KIRSTEN MÜLLER14, CHRISTOPHER D. NEEFUS10, MARIANA CABRAL DE OLIVEIRA15, LINDA RYMARQUIS16, ALISON SMITH17, JOHN W. STILLER18, WEN-KAI WU19, CHARLES YARISH20, YUN ZHUANG12, AND SUSAN H. BRAWLEY4 1Department of Cell Biology and Molecular Genetics, University of Maryland, College Park, MD 20742 2Department of Geophysics, Stanford University, Stanford, CA 94305 3 Department of Ecology, Evolution and Natural Resources, Rutgers University, New Brunswick, NJ 08901 4Department of Marine Sciences, University of Maine, Orono, ME 04469 5Department of Botany, The Natural History Museum, London, UK 6CNRS, Université Pierre et Marie Curie, Station Biologique, Roscoff, cedex, France 7Department of Plant Biology, The Carnegie Institution, Stanford, CA 94305 8Department of Chemistry, University of California, Los Angeles, CA 90095 9Algal Genetics and Chemistry, Fisheries Sciences, Hokkaido University, Hakodate, Hokkaido, Japan 10Department of Biological Sciences, University of New Hampshire, Durham, NH 03824 11 Natural and Applied Sciences, University of Southern Maine, Lewiston, ME 04240 12Department of Marine Sciences, University of Connecticut, Groton, CT 06340 13School of Life Sciences, Nanjing University, Nanjing, CN 14Department of Biology, University of Waterloo, Waterloo, Ontario, CA N2L 3G1 15Department of Botany, Biosciences Institute, University of São Paulo, São Paulo, Brazil 16Biotechnology Institute, University of Delaware, Newark, DE 19711 131 132 ELISABETH GANTT ET AL. -
Primary Characterization of a Life-Cycle Mutant Akasusabi of the Red Alga Neopyropia Yezoensis
Article Primary Characterization of a Life-Cycle Mutant akasusabi of the Red Alga Neopyropia yezoensis Koji Mikami 1,* , Takaharu Matsumura 2,3 and Yuji Yamamoto 2,4 1 Department of Food Resource Development, School of Food Industrial Sciences, Miyagi University, 2-2-1 Hatatate, Taihaku-ku, Sendai 982-0215, Japan 2 Marine Resources Research Center of Aichi Fisheries Research Institute, 2-1 Toyoura, Toyohama, Minamichita-cho, Chita 470-3412, Japan; [email protected] (T.M.); [email protected] (Y.Y.) 3 Aichi Fisheries Research Institute, 97 Wakamiya, Miya-cho, Gamagori 443-0021, Japan 4 Fisheries Administration Division, Aichi Prefectural Government, 3-1-2 Sannomaru, Naka-ku, Nagoya 460-8501, Japan * Correspondence: [email protected] Abstract: Gametophyte-to-sporophyte transition in the haploid-diploid life cycle depends on fertil- ization of male and female gametes. We describe here a mutant of the marine red seaweed Neopyropia yezoensis, designated akasusabi (aks), where the gametophyte-to-sporophyte transition occurs indepen- dently of fertilization. Although conchocelis filaments were produced from carpospores, severe defects in the maturation of carposporangia via mitosis to generate conchospores were observed. In the aks mutant, however, somatic cells of gametophytic thalli were able to produce conchocelis filaments without fertilization. Thus, apogamy occurs in aks. In addition, aks was highly sensitive to wounding that promotes both asexual and apogamous reproductive responses by producing spores, which develop either into blades or conchocelis filaments, indicating that aks responds to wounding by Citation: Mikami, K.; Matsumura, T.; enhanced reproduction. These findings indicated that the aks mutation enables the transformation of Yamamoto, Y.