Transcriptomic Analysis of Cadmium Stressed Tamarix Hispida Revealed Novel Transcripts and the Importance of ABA Network
Total Page:16
File Type:pdf, Size:1020Kb
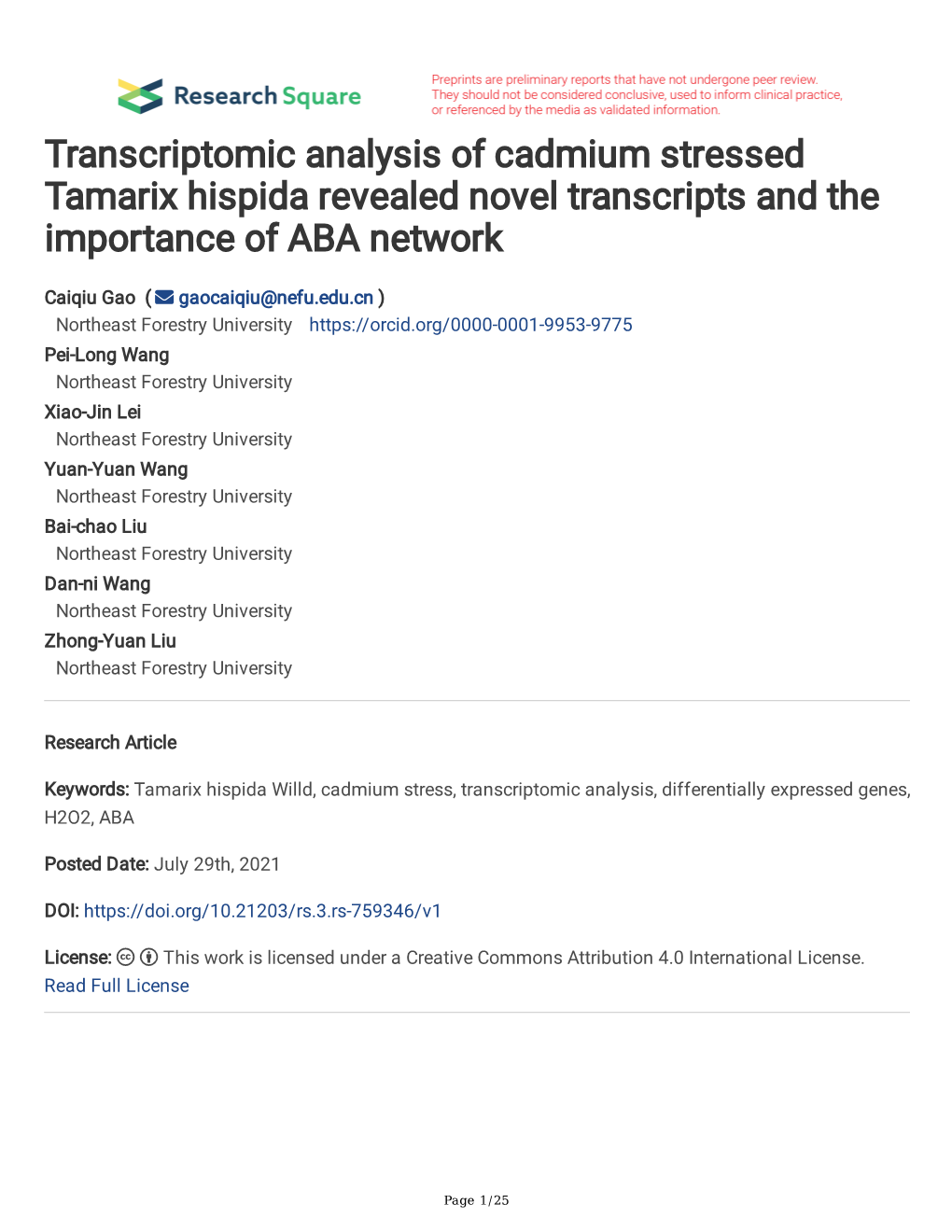
Load more
Recommended publications
-
ABA Crosstalk with Ethylene and Nitric Oxide in Seed Dormancy and Germination Erwann Arc, Julien Sechet, Françoise Corbineau, Loïc Rajjou, Annie Marion-Poll
ABA crosstalk with ethylene and nitric oxide in seed dormancy and germination Erwann Arc, Julien Sechet, Françoise Corbineau, Loïc Rajjou, Annie Marion-Poll To cite this version: Erwann Arc, Julien Sechet, Françoise Corbineau, Loïc Rajjou, Annie Marion-Poll. ABA crosstalk with ethylene and nitric oxide in seed dormancy and germination. Frontiers in Plant Science, Frontiers, 2013, 4 (63), pp.1-19. 10.3389/fpls.2013.00063. hal-01204075 HAL Id: hal-01204075 https://hal.archives-ouvertes.fr/hal-01204075 Submitted on 29 May 2020 HAL is a multi-disciplinary open access L’archive ouverte pluridisciplinaire HAL, est archive for the deposit and dissemination of sci- destinée au dépôt et à la diffusion de documents entific research documents, whether they are pub- scientifiques de niveau recherche, publiés ou non, lished or not. The documents may come from émanant des établissements d’enseignement et de teaching and research institutions in France or recherche français ou étrangers, des laboratoires abroad, or from public or private research centers. publics ou privés. REVIEW ARTICLE published: 26 March 2013 doi: 10.3389/fpls.2013.00063 ABA crosstalk with ethylene and nitric oxide in seed dormancy and germination Erwann Arc1,2, Julien Sechet1, Françoise Corbineau3, Loïc Rajjou1,2 and Annie Marion-Poll1* 1 Institut Jean-Pierre Bourgin (UMR1318 INRA – AgroParisTech), Institut National de la Recherche Agronomique, Saclay Plant Science, Versailles, France 2 UFR de Physiologie végétale, AgroParisTech, Paris, France 3 Germination et Dormance des Semences, UR5 UPMC-EAC 7180 CNRS, Université Pierre et Marie Curie-Paris 6, Paris, France Edited by: Dormancy is an adaptive trait that enables seed germination to coincide with favorable Sergi Munné-Bosch, University of environmental conditions. -
Diverse Biosynthetic Pathways and Protective Functions Against Environmental Stress of Antioxidants in Microalgae
plants Review Diverse Biosynthetic Pathways and Protective Functions against Environmental Stress of Antioxidants in Microalgae Shun Tamaki 1,* , Keiichi Mochida 1,2,3,4 and Kengo Suzuki 1,5 1 Microalgae Production Control Technology Laboratory, RIKEN Baton Zone Program, Yokohama 230-0045, Japan; [email protected] (K.M.); [email protected] (K.S.) 2 RIKEN Center for Sustainable Resource Science, Yokohama 230-0045, Japan 3 Kihara Institute for Biological Research, Yokohama City University, Yokohama 230-0045, Japan 4 School of Information and Data Sciences, Nagasaki University, Nagasaki 852-8521, Japan 5 euglena Co., Ltd., Tokyo 108-0014, Japan * Correspondence: [email protected]; Tel.: +81-45-503-9576 Abstract: Eukaryotic microalgae have been classified into several biological divisions and have evo- lutionarily acquired diverse morphologies, metabolisms, and life cycles. They are naturally exposed to environmental stresses that cause oxidative damage due to reactive oxygen species accumulation. To cope with environmental stresses, microalgae contain various antioxidants, including carotenoids, ascorbate (AsA), and glutathione (GSH). Carotenoids are hydrophobic pigments required for light harvesting, photoprotection, and phototaxis. AsA constitutes the AsA-GSH cycle together with GSH and is responsible for photooxidative stress defense. GSH contributes not only to ROS scavenging, but also to heavy metal detoxification and thiol-based redox regulation. The evolutionary diversity of microalgae influences the composition and biosynthetic pathways of these antioxidants. For example, α-carotene and its derivatives are specific to Chlorophyta, whereas diadinoxanthin and fucoxanthin are found in Heterokontophyta, Haptophyta, and Dinophyta. It has been suggested that Citation: Tamaki, S.; Mochida, K.; Suzuki, K. Diverse Biosynthetic AsA is biosynthesized via the plant pathway in Chlorophyta and Rhodophyta and via the Euglena Pathways and Protective Functions pathway in Euglenophyta, Heterokontophyta, and Haptophyta. -
Phytochemical Functional Foods Related Titles from Woodhead’S Food Science, Technology and Nutrition List
Phytochemical functional foods Related titles from Woodhead’s food science, technology and nutrition list: Performance functional foods (ISBN 1 85573 671 3) Some of the newest and most exciting developments in functional foods are products that claim to influence mood and enhance both mental and physical performance. This important collection reviews the range of ingredients used in these ‘performance’ functional foods, their effects and the evidence supporting their functional benefits. Antioxidants in food (ISBN 1 85573 463 X) Antioxidants are an increasingly important ingredient in food processing, as they inhibit the development of oxidative rancidity in fat-based foods, particularly meat and dairy products and fried foods. Recent research suggests that they play a role in limiting cardiovascular disease and cancers. This book provides a review of the functional role of antioxidants and discusses how they can be effectively exploited by the food industry, focusing on naturally occurring antioxidants in response to the increasing consumer scepticism over synthetic ingredients. ‘An excellent reference book to have on the shelves’ LWT Food Science and Technology Natural antimicrobials for the minimal processing of foods (ISBN 1 85573 669 1) Consumers demand food products with fewer synthetic additives but increased safety and shelf-life. These demands have increased the importance of natural antimicrobials which prevent the growth of pathogenic and spoilage micro-organisms. Edited by a leading expert in the field, this important collection reviews the range of key antimicrobials such as nisin and chitosan, applications in such areas as postharvest storage of fruits and vegetables, and ways of combining antimicrobials with other preservation techniques to enhance the safety and quality of foods. -
Relating Metatranscriptomic Profiles to the Micropollutant
1 Relating Metatranscriptomic Profiles to the 2 Micropollutant Biotransformation Potential of 3 Complex Microbial Communities 4 5 Supporting Information 6 7 Stefan Achermann,1,2 Cresten B. Mansfeldt,1 Marcel Müller,1,3 David R. Johnson,1 Kathrin 8 Fenner*,1,2,4 9 1Eawag, Swiss Federal Institute of Aquatic Science and Technology, 8600 Dübendorf, 10 Switzerland. 2Institute of Biogeochemistry and Pollutant Dynamics, ETH Zürich, 8092 11 Zürich, Switzerland. 3Institute of Atmospheric and Climate Science, ETH Zürich, 8092 12 Zürich, Switzerland. 4Department of Chemistry, University of Zürich, 8057 Zürich, 13 Switzerland. 14 *Corresponding author (email: [email protected] ) 15 S.A and C.B.M contributed equally to this work. 16 17 18 19 20 21 This supporting information (SI) is organized in 4 sections (S1-S4) with a total of 10 pages and 22 comprises 7 figures (Figure S1-S7) and 4 tables (Table S1-S4). 23 24 25 S1 26 S1 Data normalization 27 28 29 30 Figure S1. Relative fractions of gene transcripts originating from eukaryotes and bacteria. 31 32 33 Table S1. Relative standard deviation (RSD) for commonly used reference genes across all 34 samples (n=12). EC number mean fraction bacteria (%) RSD (%) RSD bacteria (%) RSD eukaryotes (%) 2.7.7.6 (RNAP) 80 16 6 nda 5.99.1.2 (DNA topoisomerase) 90 11 9 nda 5.99.1.3 (DNA gyrase) 92 16 10 nda 1.2.1.12 (GAPDH) 37 39 6 32 35 and indicates not determined. 36 37 38 39 S2 40 S2 Nitrile hydration 41 42 43 44 Figure S2: Pearson correlation coefficients r for rate constants of bromoxynil and acetamiprid with 45 gene transcripts of ECs describing nucleophilic reactions of water with nitriles. -
Efficient Heterologous Transformation of Chlamydomonas Reinhardtii Npq2
Mar. Drugs 2012, 10, 1955-1976; doi:10.3390/md10091955 OPEN ACCESS Marine Drugs ISSN 1660-3397 www.mdpi.com/journal/marinedrugs Article Efficient Heterologous Transformation of Chlamydomonas reinhardtii npq2 Mutant with the Zeaxanthin Epoxidase Gene Isolated and Characterized from Chlorella zofingiensis Inmaculada Couso, Baldo F. Cordero, María Ángeles Vargas and Herminia Rodríguez * Institute of Plant Biochemistry and Photosynthesis, CIC Cartuja, University of Seville and CSIC, Avda. Américo Vespucio no. 49, 41092-Seville, Spain; E-Mails: [email protected] (I.C.); [email protected] (B.F.C.); [email protected] (M.A.V.) * Author to whom correspondence should be addressed; E-Mail: [email protected]; Tel.: +34-954-489-512; Fax: +34-954-460-065. Received: 4 July 2012; in revised form: 6 August 2012 / Accepted: 22 August 2012 / Published: 12 September 2012 Abstract: In the violaxanthin cycle, the violaxanthin de-epoxidase and zeaxanthin epoxidase catalyze the inter-conversion between violaxanthin and zeaxanthin in both plants and green algae. The zeaxanthin epoxidase gene from the green microalga Chlorella zofingiensis (Czzep) has been isolated. This gene encodes a polypeptide of 596 amino acids. A single copy of Czzep has been found in the C. zofingiensis genome by Southern blot analysis. qPCR analysis has shown that transcript levels of Czzep were increased after zeaxanthin formation under high light conditions. The functionality of Czzep gene by heterologous genetic complementation in the Chlamydomonas mutant npq2, which lacks zeaxanthin epoxidase (ZEP) activity and accumulates zeaxanthin in all conditions, was analyzed. The Czzep gene was adequately inserted in the pSI105 vector and expressed in npq2. -
ABA Crosstalk with Ethylene and Nitric Oxide in Seed Dormancy and Germination
REVIEW ARTICLE published: 26 March 2013 doi: 10.3389/fpls.2013.00063 ABA crosstalk with ethylene and nitric oxide in seed dormancy and germination Erwann Arc1,2, Julien Sechet1, Françoise Corbineau3, Loïc Rajjou1,2 and Annie Marion-Poll1* 1 Institut Jean-Pierre Bourgin (UMR1318 INRA – AgroParisTech), Institut National de la Recherche Agronomique, Saclay Plant Science, Versailles, France 2 UFR de Physiologie végétale, AgroParisTech, Paris, France 3 Germination et Dormance des Semences, UR5 UPMC-EAC 7180 CNRS, Université Pierre et Marie Curie-Paris 6, Paris, France Edited by: Dormancy is an adaptive trait that enables seed germination to coincide with favorable Sergi Munné-Bosch, University of environmental conditions. It has been clearly demonstrated that dormancy is induced by Barcelona, Spain abscisic acid (ABA) during seed development on the mother plant. After seed dispersal, Reviewed by: germination is preceded by a decline in ABA in imbibed seeds, which results from ABA Inhwan Hwang, Pohang University of Science and Technology, South Korea catabolism through 8 -hydroxylation. The hormonal balance between ABA and gibberellins Dawei Yan, University of Toronto, (GAs) has been shown to act as an integrator of environmental cues to maintain dormancy Canada or activate germination. The interplay of ABA with other endogenous signals is however *Correspondence: less documented. In numerous species, ethylene counteracts ABA signaling pathways Annie Marion-Poll, Institut Jean-Pierre and induces germination. In Brassicaceae seeds, ethylene prevents the inhibitory effects Bourgin (UMR1318 INRA – AgroParisTech), Institut National de la of ABA on endosperm cap weakening, thereby facilitating endosperm rupture and radicle Recherche Agronomique, Saclay Plant emergence. Moreover, enhanced seed dormancy in Arabidopsis ethylene-insensitive Science, Route de Saint Cyr, F-78026 mutants results from greater ABA sensitivity. -
Source: the Arabidopsis Information Resource (TAIR);
Table S1 List of targeted loci and information about their function in Arabidopsis thaliana (source: The Arabidopsis Information Resource (TAIR); https://www.arabidopsis.org/tools/bulk/genes/index.jsp). Locus Gene Model Gene Model Description Gene Model Primary Gene Symbol All Gene Symbols Identifier Name Type AT1G78800 AT1G78800.1 UDP-Glycosyltransferase superfamily protein_coding protein;(source:Araport11) AT5G06830 AT5G06830.1 hypothetical protein;(source:Araport11) protein_coding AT2G31740 AT2G31740.1 S-adenosyl-L-methionine-dependent methyltransferases protein_coding superfamily protein;(source:Araport11) AT5G11960 AT5G11960.1 magnesium transporter, putative protein_coding (DUF803);(source:Araport11) AT4G00560 AT4G00560.4 NAD(P)-binding Rossmann-fold superfamily protein_coding protein;(source:Araport11) AT1G80510 AT1G80510.1 Encodes a close relative of the amino acid transporter ANT1 protein_coding (AT3G11900). AT2G21250 AT2G21250.1 NAD(P)-linked oxidoreductase superfamily protein_coding protein;(source:Araport11) AT5G04420 AT5G04420.1 Galactose oxidase/kelch repeat superfamily protein_coding protein;(source:Araport11) AT4G34910 AT4G34910.1 P-loop containing nucleoside triphosphate hydrolases protein_coding superfamily protein;(source:Araport11) AT5G66120 AT5G66120.2 3-dehydroquinate synthase;(source:Araport11) protein_coding AT1G45110 AT1G45110.1 Tetrapyrrole (Corrin/Porphyrin) protein_coding Methylase;(source:Araport11) AT1G67420 AT1G67420.2 Zn-dependent exopeptidases superfamily protein_coding protein;(source:Araport11) AT3G62370 -
The Health Benefits of Fruits and Vegetables
The Health Benefits of Fruits and Vegetables • Mercedes Del Río Celestino and Rafael Villa Font The Health Benefits of Fruits and Vegetables Edited by Mercedes Del Río Celestino and Rafael Font Villa Printed Edition of the Special Issue Published in Foods www.mdpi.com/journal/foods The Health Benefits of Fruits and Vegetables The Health Benefits of Fruits and Vegetables Special Issue Editors Mercedes Del R´ıoCelestino Rafael Font Villa MDPI • Basel • Beijing • Wuhan • Barcelona • Belgrade • Manchester • Tokyo • Cluj • Tianjin Special Issue Editors Mercedes Del R´ıo Celestino Rafael Font Villa Agri-food Laboratory of Córdoba Agri-food Laboratory of Córdoba CAGPDS, Junta de Andalucía CAGPDS, Junta de Andalucía Córdoba, Spain Córdoba, Spain Editorial Office MDPI St. Alban-Anlage 66 4052 Basel, Switzerland This is a reprint of articles from the Special Issue published online in the open access journal Foods (ISSN 2304-8158) (available at: https://www.mdpi.com/journal/foods/special issues/Fruits phytochemicals Epidemiological). For citation purposes, cite each article independently as indicated on the article page online and as indicated below: LastName, A.A.; LastName, B.B.; LastName, C.C. Article Title. Journal Name Year, Article Number, Page Range. ISBN 978-3-03928-829-8 (Hbk) ISBN 978-3-03928-830-4 (PDF) Cover image courtesy of Mercedes del R´ıo Celestino. c 2020 by the authors. Articles in this book are Open Access and distributed under the Creative Commons Attribution (CC BY) license, which allows users to download, copy and build upon published articles, as long as the author and publisher are properly credited, which ensures maximum dissemination and a wider impact of our publications. -
Chili Pepper Carotenoids: Nutraceutical Properties and Mechanisms of Action
molecules Review Chili Pepper Carotenoids: Nutraceutical Properties and Mechanisms of Action Maria Guadalupe Villa-Rivera and Neftalí Ochoa-Alejo * Departamento de Ingeniería Genética, Centro de Investigación y de Estudios Avanzados del Instituto Politécnico Nacional, Unidad Irapuato, Guanajuato 36824, Mexico; [email protected] * Correspondence: [email protected] Academic Editor: Karel Šmejkal Received: 23 October 2020; Accepted: 21 November 2020; Published: 27 November 2020 Abstract: Chili pepper is a prominent cultivated horticultural crop that is traditionally used for food seasoning and is applied for the treatment and prevention of multiple diseases. Its beneficial health properties are due to its abundance and variety of bioactive components, such as carotenoids, capsaicinoids, and vitamins. In particular, carotenoids have important nutraceutical properties, and several studies have focused on their potential in the prevention and treatment of human diseases. In this article, we reviewed the state of knowledge of general aspects of chili pepper carotenoids (biosynthesis pathway, types and content in Capsicum spp., and the effects of processing on carotenoid content) and recent findings on the effects of carotenoid nutraceuticals, such as antioxidant, cancer preventive, anti-inflammatory, cardiovascular disorder preventive, and anti-obesity effects. Keywords: Capsicum; carotenoids; chili pepper; nutraceutical effects; antioxidant; cancer; cardiovascular disorders; anti-inflammatory; obesity 1. Introduction Chili pepper (Genus Capsicum, Family Solanaceae) is an important cultivated spice crop. During 2018, 4.2 million tons of dry chilies and peppers and 36.8 million tons of green chilies and ≈ ≈ peppers were produced worldwide (FAOSTATS 2018) [1]. The Capsicum genus comprises 38 different species, but only C. annuum, C. frutescens, C. chinense, C. baccatum, and C. -
Genomic Adaptations of the Green Alga Dunaliella Salina to Life Under High Salinity
Genomic adaptations of the green alga Dunaliella salina to life under high salinity. Jürgen E.W. Polle1,2,3* Sara Calhoun3 Zaid McKie-Krisberg1,4 Simon Prochnik3,5 Peter Neofotis6 Won C. Yim7 Leyla T. Hathwaik7 Jerry Jenkins3,8 Henrik Molina9 Jakob Bunkenborg10 Igor GrigorieV3,11 Kerrie Barry3 Jeremy Schmutz3,8 EonSeon Jin12 John C. Cushman7 Jon K. Magnusson13 1Department of Biology, Brooklyn College of the City UniVersity of New York, Brooklyn, NY 11210, USA 2The Graduate Center of the City UniVersity of New York, New York, NY 10016 USA 3U.S. Department of Energy Joint Genome Institute, Lawrence Berkeley National Laboratory, Berkeley, CA 94720, USA 4Current address: Department of Information SerVices and Technology, SUNY Downstate Health Sciences UniVersity, Brooklyn, NY 11203, USA 5Current address: MBP Titan LLC, South San Francisco, CA 94080, USA 6Current address: U.S. Department of Energy – Plant Research Laboratory, Michigan State UniVersity, East Lansing, MI, 48824 USA 7UniVersity of NeVada, Department of Biochemistry and Molecular Biology, Reno, NeVada, USA 8HudsonAlpha Institute for Biotechnology, HuntsVille, Alabama, USA 9The Proteomics Resource Center, The Rockefeller UniVersity, New York, New York, USA 10Alphalyse A/S, Odense, Denmark 11Department of Plant and Microbial Biology, UniVersity of California - Berkeley, 111 Koshland Hall, Berkeley, CA 94720, USA 12Department of Life Science, Hanyang UniVersity, Research Institute for Natural Sciences, Seoul, Republic of Korea 13Pacific Northwest National Laboratory, Richland, Washington, USA * Corresponding author: Dr. Jürgen E.W. Polle, [email protected] 1 Abstract Life in high salinity enVironments poses challenges to cells in a Variety of ways: maintenance of ion homeostasis and nutrient acquisition, often while concomitantly enduring saturating irradiances. -
A Review of the Biological Activities of Microalgal Carotenoids and Their Potential Use in Healthcare and Cosmetic Industries
marine drugs Review A Review of the Biological Activities of Microalgal Carotenoids and Their Potential Use in Healthcare and Cosmetic Industries Ramaraj Sathasivam ID and Jang-Seu Ki * Department of Biotechnology, Sangmyung University, Seoul 03016, Korea; [email protected] * Correspondence: [email protected]; Tel.: +82-2-2287-5449; Fax: +82-2-2287-0070 Received: 17 November 2017; Accepted: 8 January 2018; Published: 12 January 2018 Abstract: Carotenoids are natural pigments that play pivotal roles in many physiological functions. The characteristics of carotenoids, their effects on health, and the cosmetic benefits of their usage have been under investigation for a long time; however, most reviews on this subject focus on carotenoids obtained from several microalgae, vegetables, fruits, and higher plants. Recently, microalgae have received much attention due to their abilities in producing novel bioactive metabolites, including a wide range of different carotenoids that can provide for health and cosmetic benefits. The main objectives of this review are to provide an updated view of recent work on the health and cosmetic benefits associated with carotenoid use, as well as to provide a list of microalgae that produce different types of carotenoids. This review could provide new insights to researchers on the potential role of carotenoids in improving human health. Keywords: carotenoids; microalgae; anti-angiogenic; cardioprotective activity; anti-cancer; anti-diabetic; anti-inflammatory; anti-obesity; anti-oxidant; beauty 1. Introduction Carotenoids have a wide range of applications in the healthcare and nutraceuticals industry. The growing importance of carotenoid use in improving food quality has also led to an increase in the demand for carotenoids in the global market. -
Substrate Specificity and Reaction Mechanism of Vertebrate Carotenoid Cleavage
Substrate specificity and reaction mechanism of vertebrate carotenoid cleavage oxygenases DISSERTATION Presented in Partial Fulfillment of the Requirements for the Degree Doctor of Philosophy in the Graduate School of The Ohio State University By Carlo C. dela Seña The Ohio State Biochemistry Program The Ohio State University 2014 Dissertation Committee: Earl H. Harrison, Ph.D., Adviser Robert W. Curley, Jr., Ph.D. Ross E. Dalbey, Ph.D. Steven J. Schwartz, Ph.D Copyright by Carlo C. dela Seña 2014 i Abstract Carotenoids are yellow, orange and red pigments found in fruits and vegetables. Some carotenoids can act as dietary precursors of vitamin A. Humans and other animals generate retinal (vitamin A aldehyde) from provitamin A carotenoids by oxidative cleavage of the central 15-15′ double bond by the enzyme β-carotene 15-15′-oxygenase (BCO1). Another carotenoid oxygenase, β-carotene 9′-10′-oxygenase (BCO2), catalyzes the oxidative cleavage of the 9′-10′ double bond of various carotenoids to yield apo-10′- carotenals and ionones. In this dissertation, we elucidate the substrate specificity of these two enzymes. Recombinant His-tagged human BCO1 was expressed in Escherichia coli strain BL21- Gold (DE3) and purified by cobalt ion affinity chromatography. The enzyme was incubated with various dietary carotenoids and β-apocarotenals, and the reaction products were analyzed by reverse-phase high-performance liquid chromatography (HPLC). We found that BCO1 catalyzes the oxidative cleavage of only provitamin A dietary carotenoids and β-apocarotenals specifically at the 15-15′ double bond to yield retinal. A notable exception is lycopene, which is cleaved by BCO1 to yield two molecules of acycloretinal.