Environmental Geochemistry of Radioactive Contamination
Total Page:16
File Type:pdf, Size:1020Kb
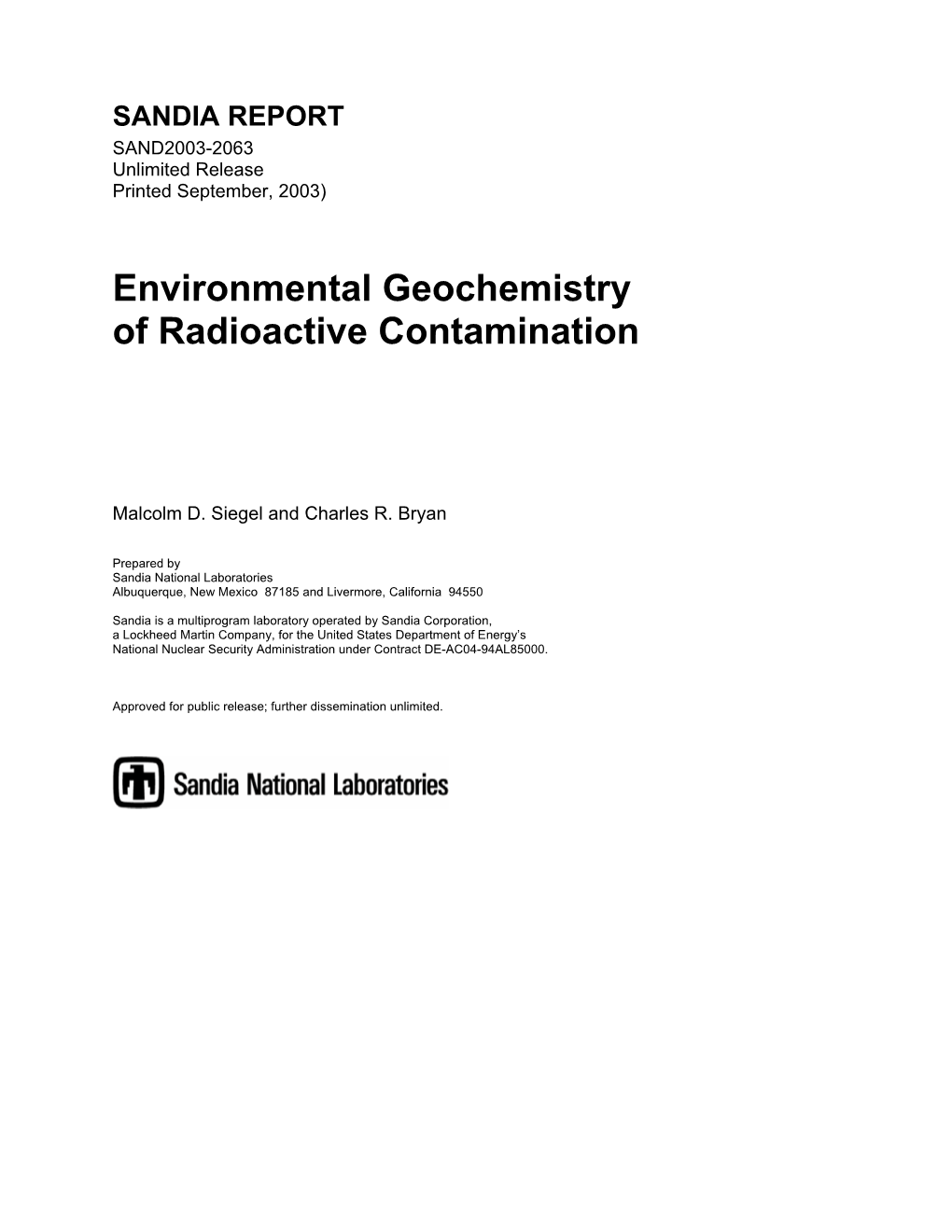
Load more
Recommended publications
-
Security Operational Skills 2 (Tracing).P65
Unit - 4 K Operating Skill for handling Natural Disasters Structure 4.1 Objectives 4.2 Introduction 4.3 Operating Skill for natural and nuclear disasters 4.4 Accident Categories 4.5 Nuclear and radiation accidents and incidents 4.6 Geological disasters 4.7 Operating Skills for handling Mines and other Explosive Devices 4.8 Operating Skills for handing hijacking situation (other than an airline hijacking 4.9 Operating skills for antivehicle theft operations 4.10 Operating skills for facing a kidnapping or hostage situation 4.11 Operating Skill for handling coal mines and other explosive devices 4.12 Hostage Rights : Law and Practice in Throes of Evolution 4.12.1 Terminology 4.13 Relative Value of Rights 4.14 Conflict of Rights and Obligations 4.15 Hong Kong mourns victims of bus hijacking in the Philoppines 4.16 Rules for Successful Threat Intelligence Teams 4.16.1 Tailor Your Talent 4.16.2 Architect Your Infrastructure 4.16.3 Enable Business Profitability 4.16.4 Communicate Continuously 4.17 Construction Safety Practices 4.17.1 Excavation 4.17.2 Drilling and Blasting 4.17.3 Piling and deep foundations 234 4.18 Planning 4.18.1 Steps in Planning Function 4.18.2 Characteristics of planning 4.18.3 Advantages of planning 4.18.4 Disadvantages of planning 4.1 Objectives The following is a list of general objectives departments should consider when creating an Information Disaster Prevention and Recovery Plan: O Ensure the safety of all employees and visitors at the site/facility O Protect vital information and records O Secure business sites -
Chernobyl Template.Qxd 16/09/2019 11:08 Page 39
9Chernobyl_Template.qxd 16/09/2019 11:08 Page 39 39 Chernobyl On 3 February 1987, during a lecture trip to Japan, I was invited to meet five members of the Japan Atomic Industrial Forum Inc. Zhores Medvedev They wanted to discuss my book, Nuclear Disaster in the Urals, which described the consequences of the Kyshtym disaster, an On 8 August 2019, a explosion at a nuclear waste site in the deadly nuclear explosion Soviet Union in 1957. took place in northern The book, published in New York in 1979 Russia in the vicinity of and translated into Japanese in 1982, was the Nenoksa weapons then still the only published description of testing range. At least five this accident. The Kyshtym disaster was not people are said to have yet included in a list of nuclear accidents died. Subsequently, a prepared by the International Atomic Russian state weather Energy Agency (IAEA). Top of this list, agency confirmed release recorded at a topofthescale 7 in severity, into the atmosphere of was Chernobyl, Three Mile Island was strontium, barium and scale 5, and the fire at Windscale in other radioactive isotopes, England, in 1957, was scale 3. (The indicating that a nuclear International Nuclear Event Scale was reactor was involved in revised several times, subsequently, and the the explosion. fire at Windscale is now reckoned to be Zhores Medvedev died scale 5.) in 2018, before this recent In 1987, I had already started to study the explosion. Back in 2011, available information on Chernobyl he charted a trail of because I was not satisfied with the Soviet nuclear disasters from Report to the IAEA, which blamed mainly Kyshtym in the the power station personnel for gross Cheliabinsk region of operational errors. -
Benefit-Function of Two- Identical Cold Standby Nuclear Reactors System Subject to Failure Due to Radioactivity Or Overheating, Steam Explosion, Fire, and Meltdown
International Journal on Mechanical Engineering and Robotics (IJMER) ________________________________________________________________________________________________ Benefit-Function of Two- Identical Cold Standby Nuclear Reactors System subject to failure due to radioactivity or Overheating, steam explosion, fire, and meltdown Ashok Kumar Saini BLJS COLLEGE, TOSHAM (BHIWANI) HARYANA INDIA Email ID [email protected] The accident killed 30 people directly and damaged Abstract- A nuclear and radiation accident is defined by approximately $7 billion of property. A study published in the International Atomic Energy Agency as "an event that 2005 estimates that there will eventually be up to 4,000 has led to significant consequences to people, the additional cancer deaths related to the accident among environment or the facility." Examples include lethal those exposed to significant radiation levels. Radioactive effects to individuals, large radioactivity release to fallout from the accident was concentrated in areas of the environment, or reactor core melt." The prime Belarus, Ukraine and Russia. Approximately 350,000 example of a "major nuclear accident" is one in which people were forcibly resettled away from these areas soon a reactor core is damaged and significant amounts after the accident. of radioactivity are released, such as in the Chernobyl disaster in 1986. Benjamin K. Sovacool has reported that worldwide there have been 99 accidents at nuclear power plants from 1952 The impact of nuclear accidents has been a topic of debate to 2009 (defined as incidents that either resulted in the loss practically since the first nuclear reactors were constructed of human life or more than US$50,000 of property damage, in 1954. It has also been a key factor in public concern the amount the US federal government uses to define about nuclear facilities. -
18.06.01 Status Report 2017
Decommissioning Russia’s old nuclear power reactors: Status update on key processes 2017 The report is made in the frame of the project “From closed rooms to openness”, financially supported by the Norwegian Radiation Protection Authority over the Nuclear Action Plan. Project partners from Russia, Ukraine and Norway cooperate to promote safe, social and environmental acceptable decommissioning of old nuclear power plant reactors, including handling of radioactive waste and spent nuclear fuel. We believe in openness and participation of all stakeholders in the decision-making processes, including authorities at all levels, business and civil society. Published by • Russian Social-Ecological Union / Friends of the Earth Russia (Russia) • Kola Environmental Center (Apatity, Murmansk Region, Russia) • Public Council of the South Coast of the Gulf of Finland (St. Petersburg – Leningrad Oblast, Russia) • Za Prirodu/ For Nature (Chelyabinsk, Russia) • Naturvernforbundet/ Friends of the Earth Norway (Norway) Edited by Kjersti Album, Naturvernforbundet/Friends of the Earth Norway Contributions by Oleg Bodrov, Yuri Ivanov, Dag Arne Høystad, Daria Matveenkova, Olga Senova, Vitaly Servetnik, Andrey Talevlin Front page design Kristine Kleppo For more information, please contact the participating partners directly or refer to our reports, which can be found at the pages of Russian Social-Ecological Union: http://rusecounion.ru/decomatom St.Petersburg, Sosnovy Bor, Chelyabinsk, Apatity/Murmansk, Oslo – May 2018 2 Decommissioning Russia’s old nuclear power reactors: Status update on key processes 2017 Decommissioning Russia’s old nuclear power reactors Status update on key processes 2017 3 Decommissioning Russia’s old nuclear power reactors: Status update on key processes 2017 Content 1. Introduction 4 2. -
The Plutonium Challenge-Environmental Issues
Plutonium Overview The Plutonium Challenge Environmental issues he environmental concerns about plutonium stem from its potentially harmful effects on human health. Unlike many industrial materials whose Ttoxicity was discovered only after years of use, plutonium was immediately recognized as dangerous and as requiring special handling care. Consequently, the health effects on plutonium workers in the United States and the general public have been remarkably benign. Nevertheless, the urgency of the wartime effort and the intensity of the arms race during the early years of the Cold War resulted in large amounts of radioactivity being released into the environment in the United States and Russia. These issues are being addressed now, especially in the United States. Science and international cooperation will play a large role in minimizing the potential health effects on future generations. Environmental Consequences of the Cold War The environmental problems resulting from wartime and Cold War nuclear operations were for the most part kept out of public view during the arms race between the United States and the Soviet Union. On the other hand, concerns over health effects from atmospheric testing were debated during the 1950s, leading to the 1963 Limited Test Ban Treaty, which banned nuclear testing everywhere except underground. The U.S. nuclear weapons complex was not opened for public scrutiny until the late 1980s, following a landmark court decision on mercury cont- amination at the Oak Ridge, Tennessee, facilities of the Department of Energy (DOE) in 1984. In the Soviet Union, all nuclear matters, including environmental problems in the nuclear weapons complex, were kept secret. -
Subsurface Behavior of Plutonium and Americium at Non-Hanford Sites and Relevance to Hanford
PNNL-17386 Subsurface Behavior of Plutonium and Americium at Non-Hanford Sites and Relevance to Hanford KJ Cantrell RG Riley February 2008 DISCLAIMER This report was prepared as an account of work sponsored by an agency of the United States Government. Neither the United States Government nor any agency thereof, nor Battelle Memorial Institute, nor any of their employees, makes any warranty, express or implied, or assumes any legal liability or responsibility for the accuracy, completeness, or usefulness of any information, apparatus, product, or process disclosed, or represents that its use would not infringe privately owned rights. Reference herein to any specific commercial product, process, or service by trade name, trademark, manufacturer, or otherwise does not necessarily constitute or imply its endorsement, recommendation, or favoring by the United States Government or any agency thereof, or Battelle Memorial Institute. The views and opinions of authors expressed herein do not necessarily state or reflect those of the United States Government or any agency thereof. PACIFIC NORTHWEST NATIONAL LABORATORY operated by BATTELLE for the UNITED STATES DEPARTMENT OF ENERGY under Contract DE-AC05-76RL01830 Printed in the United States of America Available to DOE and DOE contractors from the Office of Scientific and Technical Information, P.O. Box 62, Oak Ridge, TN 37831-0062; ph: (865) 576-8401 fax: (865) 576-5728 email: [email protected] Available to the public from the National Technical Information Service, U.S. Department of Commerce, 5285 Port Royal Rd., Springfield, VA 22161 ph: (800) 553-6847 fax: (703) 605-6900 email: [email protected] online ordering: http://www.ntis.gov/ordering.htm This document was printed on recycled paper. -
Radioactive Contamination at Chelyabinsk-65, Russia
AM". Rev. EJlerv EJlwOIt. 1993. /8:507-28 Copyrjgh' @ /993 by Annual Rrnewl III". AU righlS relerved RADIOACTIVE CONTAMINATION AT CHELYABINSK-65, RUSSIA Thomas B. Cochran, Robert Standish Norris, and Kristen L. Suokko Natural Rewurces Defense Council, Washington. DC KEY WORDS:radioactive waste. Mayak, nuclear accidents. Lake Kamchay. plutonium production INTRODUcnON _. 507 WASTE MANAGEMF.NI ACTlVmES _ _ _ _ . 510 Discharge of Waste Into the Tecba River _ . Sit Lake Karaehay (Reservoir 9) .... _ . 515 Lake Slame Bololo (Old Swamp; Reservoir 17) _ '. 519' WilSIe Explosion in 1951 ..•.............. _ . 520 WASTE MANAGEMENT TODAY • . • . • . • . 522 Storage ~ .Hi~LeveI Waste Tanks " _ _ '. 523 Waste VltnficatlOll ...•......•.............•••...•.•...... 523 Solid Waste Burial . • . 524 CONCLUSION _ . 52S APPENDIX-BASIC UNITS OF IONIZING RADIATION _ . • . 5~ Fifteen k.ilometerseast of the city of Kyshtym, on the east side of the Ural Mountains, sits the once secret complex of Chelyabinsk-65. home of the Mayak Chemical Combine. The Mayak facility housed the fonner Soviet Union's first industrial nuclear reactors, and produced the material for the country's first atomic bomb beginning in 1948. Over four decades of nuclear materials production and processing, the Mayak.facility discharged effluents containing more than 123 million curies (MCi) of long-lived radioactivity into an open storage lake and other sites, from which some millions have leaked into the general environment. Although the facility has adopted a number of new procedures for managing the waste, serious problems remain. 507 Chelyabinsk-65 did not receive foreign visitors, and was not on maps of the Soviet Union until 1989. Prior to about 1990, it was called Cbelyabinsk- 40. -
Assessment of the Nuclear Power Industry
Assessment of the Nuclear Power Industry – Final Report June 2013 Navigant Consulting, Inc. For EISPC and NARUC Funded by the U.S. Department of Energy Assessment of the Nuclear Power Industry Study 5: Assessment of the Location of New Nuclear and Uprating Existing Nuclear Whitepaper 5: Consideration of other Incentives/Disincentives for Development of Nuclear Power prepared for Eastern Interconnection States’ Planning Council and National Association of Regulatory Utility Commissioners prepared by Navigant Consulting, Inc. Navigant Consulting, Inc. 77 South Bedford Street, Suite 400 Burlington, MA 01803 781.270.0101 www.navigant.com Table of Contents Forward ....................................................................................................................................... ix Basic Nuclear Power Concepts ................................................................................................ 1 Executive Summary ................................................................................................................... 5 1. BACKGROUND .................................................................................................................... 9 1.1 Early Years – (1946-1957) ........................................................................................................................ 9 1.1.1 Shippingport ............................................................................................................................. 11 1.1.2 Power Reactor Demonstration Program .............................................................................. -
Nuclear Disaster at Mayak in 1957
Nuclear disaster at Mayak in 1957 Jan Willem Storm van Leeuwen independent consultant member of the Nuclear Consulting Group July 2019 [email protected] Note In this document the references are coded by Q-numbers (e.g. Q6). Each reference has a unique number in this coding system, which is consistently used throughout all publications by the author. In the list at the back of the document the references are sorted by Q-number. The resulting sequence is not necessarily the same order in which the references appear in the text. m13mayakdisaster20190713 1 Mayak (Kyshtym) The disaster at Mayak, also known as the Kyshtym disaster, has been kept secret for decades and is still being concealed, details are scarce. A quote from [Greenpeace 2007a] Q721 reads: The Mayak nuclear plant in the Southern Urals was one of the dark secrets of the cold war. It was the Soviet Union’s primary nuclear complex, a massive set of plutonium production reactors, fuel production facilities, and reprocessing and waste storage buildings. In 1957 a storage tank with highly radioactive liquid waste exploded. More than half the amount of radioactive waste released by the accident in Chernobyl was blasted into the atmosphere. A few villagers were evacuated, but most were not. 217 towns and at least 272,000 people were exposed to chronic levels of radiation. The plume was 50 kilometers wide and 1,000 kilometers long. But the explosion wasn’t the only incident of contamination. Between 1948 and 1956 radioactive waste was poured straight into the Techa River, the source of drinking water for many villages. -
Making the Russian Bomb from Stalin to Yeltsin
MAKING THE RUSSIAN BOMB FROM STALIN TO YELTSIN by Thomas B. Cochran Robert S. Norris and Oleg A. Bukharin A book by the Natural Resources Defense Council, Inc. Westview Press Boulder, San Francisco, Oxford Copyright Natural Resources Defense Council © 1995 Table of Contents List of Figures .................................................. List of Tables ................................................... Preface and Acknowledgements ..................................... CHAPTER ONE A BRIEF HISTORY OF THE SOVIET BOMB Russian and Soviet Nuclear Physics ............................... Towards the Atomic Bomb .......................................... Diverted by War ............................................. Full Speed Ahead ............................................ Establishment of the Test Site and the First Test ................ The Role of Espionage ............................................ Thermonuclear Weapons Developments ............................... Was Joe-4 a Hydrogen Bomb? .................................. Testing the Third Idea ...................................... Stalin's Death and the Reorganization of the Bomb Program ........ CHAPTER TWO AN OVERVIEW OF THE STOCKPILE AND COMPLEX The Nuclear Weapons Stockpile .................................... Ministry of Atomic Energy ........................................ The Nuclear Weapons Complex ...................................... Nuclear Weapon Design Laboratories ............................... Arzamas-16 .................................................. Chelyabinsk-70 -
Assessment of Various Types of Uncertainty in the Techa River
PNNL-18913 Prepared for the U.S. Department of Energy under Contract DE-AC05-76RL01830 Assessment of Uncertainty in the Radiation Doses for the Techa River Dosimetry System BA Napier LR Anspaugh MO Degteva NB Shagina October 2009 DISCLAIMER This report was prepared as an account of work sponsored by an agency of the United States Government. Neither the United States Government nor any agency thereof, nor Battelle Memorial Institute, nor any of their employees, makes any warranty, express or implied, or assumes any legal liability or responsibility for the accuracy, completeness, or usefulness of any information, apparatus, product, or process disclosed, or represents that its use would not infringe privately owned rights. Reference herein to any specific commercial product, process, or service by trade name, trademark, manufacturer, or otherwise does not necessarily constitute or imply its endorsement, recommendation, or favoring by the United States Government or any agency thereof, or Battelle Memorial Institute. The views and opinions of authors expressed herein do not necessarily state or reflect those of the United States Government or any agency thereof. PACIFIC NORTHWEST NATIONAL LABORATORY operated by BATTELLE for the UNITED STATES DEPARTMENT OF ENERGY under Contract DE-AC05-76RL01830 Printed in the United States of America Available to DOE and DOE contractors from the Office of Scientific and Technical Information, P.O. Box 62, Oak Ridge, TN 37831-0062; ph: (865) 576-8401 fax: (865) 576-5728 email: [email protected] Available to the public from the National Technical Information Service, U.S. Department of Commerce, 5285 Port Royal Rd., Springfield, VA 22161 ph: (800) 553-6847 fax: (703) 605-6900 email: [email protected] online ordering: http://www.ntis.gov/ordering.htm This document was printed on recycled paper. -
Speech-97-004:JOINT AMERICAN-RUSSIAN RADIATION
United States Nuclear Regulatory Commission Office of Public Affairs Washington, DC 20555 Phone 301-415-8200 Fax 301-415-2234 Internet:[email protected] No. S-97-04 NOTE: The graphics included in this speech may be obtained from the NRC’s Office of Public Affairs. The speech with the graphics is available on the NRC’s web site: <http://www.nrc.gov/OPA/gmo/nrarcv/s-97-04.htm>. JOINT AMERICAN-RUSSIAN RADIATION HEALTH EFFECTS RESEARCH Commissioner Greta Joy Dicus U.S. Nuclear Regulatory Commission Presentation to the joint meeting of American Nuclear Society, Washington, D.C. Section and Health Physics Society, Baltimore-Washington Chapter January 16, 1997 INTRODUCTION Thank you for inviting me to address the joint meeting of the Baltimore-Washington area chapters of the American Nuclear Society and the Health Physics Society. My subject is the unique opportunity to learn about radiation health effects from studies of Russian workers and populations in the southern Urals area of Russia who were exposed to radiation as a result of the operation of a Russian nuclear complex located in the southern Urals region. This opportunity was brought to the attention of the U.S. government in 1992 when then NRC Commissioner Gail de Planque wrote to the Department of State, stating "The political changes that have occurred in the former Soviet Union have opened up a unique opportunity to study and greatly increase our understanding of...health effects of radiation." As a result of Commissioner de Planque's initiative, the Russian Federation and the United States governments entered into an agreement to conduct joint research of radiation health effects.