Space Colonization, a Study of Supply and Demand
Total Page:16
File Type:pdf, Size:1020Kb
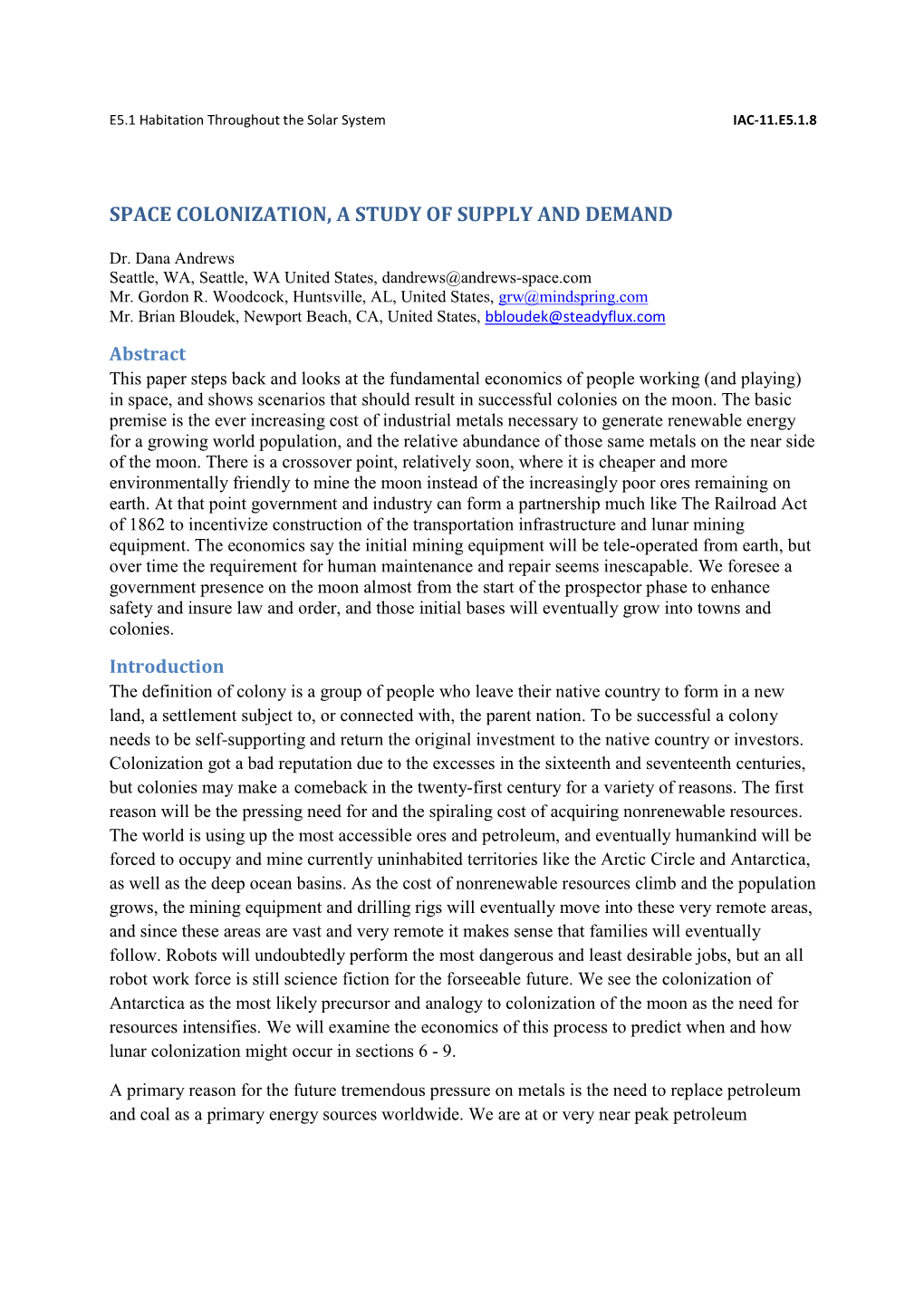
Load more
Recommended publications
-
Advanced Aluminum Powder Metallurgy Alloys and Composites
ASM Handbook, Volume 7: Powder Metal Technologies and Applications Copyright © 1998 ASM International® P.W. Lee, Y. Trudel, R. Iacocca, R.M. German, B.L. Ferguson, W.B. Eisen, K. Moyer, All rights reserved. D. Madan, and H. Sanderow, editors, p 840-858 www.asminternational.org DOI: 10.1361/asmhba0001577 Advanced Aluminum Powder Metallurgy Alloys and Composites Ram B. Bhagat, The Pennsylvania State University POWDER METALLURGICAL PROCESS- bide whisker, however, is currently the most Selection of suitable composition of the ma- ING provides much finer and homogeneous widely utilized reinforcement for the DRA com- trix material is important to meet mechanical and microstructure, better mechanical properties, posites for obtaining high resistance to creep and physical property requirements of the aluminum- and near-net shape parts producibility for alumi- higher use temperatures. Early work on whisker matrix composites. Minor alloying additions in num alloys in comparison with ingot metallurgy reinforcement started in the 1960s. Brenner (Ref the wrought alloys are generally detrimental to (I/M). In addition to the conventional blending 7, 8) and Sutton (Ref 9, 10) used tx-Al203 the mechanical properties of the composites be- and consolidation of elemental or prealloyed whiskers to fabricate metal-matrix composites cause of undesirable interfacial reaction (Ref powders into near-net shape parts, emerging (MMCs). These early composites were not at- 26-28) during the P/M consolidation. The P/M processes such as mechanical alloying and rapid tractive because of their relatively low strength route for producing the discontinuously rein- solidification (RS) create composite powders and the high cost of the whisker. -
Chromium Powder Metallurgy Steels
Technologies and Processes for the Advancement of Materials ISSUE FOCUS /// SINTERING / POWDER METALLURGY COMMERCIAL SINTERING OF CHROMIUM POWDER METALLURGY STEELS COMPANY PROFILE /// Nitrex JUNE 2020 thermalprocessing.com PERFECTION CREATED BY PRECISION. www.heat-treatment-services.com ALD is a leader in vacuum process technology and heat Global service centers treatment services. ▪ LIMBACH OBERFROHNA Precision and perfection in the pieces that we treat under our patented thermal process. ▪ GERMANY ▪ PORT HURON, MICHIGAN Leaders in the control of distortion ▪ Low Pressure Carburizing ▪ USA ▪ High pressure gas quenching Gas Nitriding ▪ RAMOS ARIZPE, COAHUILA ▪ ▪ Ferritic Nitro Carburizing ▪ MEXICO ▪ Normalizing ▪ Hardening ▪ Annealing ▪ Brazing ▪ Cryogenic Treatments ▪ Engineering services and process development Thomas Lord, Sales Director Prototype and trials United States ALD Port Huron ▪ ALD Thermal Treatment Inc. 2656 24th Street Port Huron, MI, USA 48040 Cell Number +1 (810) 300-1437 Edwin Orozco, Sales Director – Mexico Email: [email protected] Office Phone +52 844 866 9791 Cell Phone +52 1 844 277 2257 Christopher Totten, Sales – Canada / USA, [email protected] Office Phone +1 810 357 0634 [email protected] Cell Phone +1 810 300 3601 e-mail: [email protected] Moises Garcia – Sales Director - Bajio Cell Phone +521 (844) 277 2254 Mexico ALD Tratamientos Térmicos S.A. de C.V. [email protected] Our leading edge vacuum technology provides precise control and repeatability for consistently superior parts. 9001:2015 AS 9100D Vacuum Heat Treating & Brazing Services Annealing • Aging • Carburizing • Nitriding • Stress Relieving • Degassing • Brazing • Harden and Temper Sintering • Solution Treat and Age (STA) • Homogenizing • Creep Forming • Hydriding / Dehydriding Solve your toughest thermal processing challenges by utilizing our brain-trust of metallurgists, chemists and engineers. -
A Review on Selective Laser Sintering/Melting (SLS/SLM) of Aluminium Alloy Powders: Processing, Microstructure, and Properties
This is a repository copy of A review on selective laser sintering/melting (SLS/SLM) of aluminium alloy powders: Processing, microstructure, and properties. White Rose Research Online URL for this paper: http://eprints.whiterose.ac.uk/90338/ Version: Accepted Version Article: Olakanmi, EO, Cochrane, RF and Dalgarno, KW (2015) A review on selective laser sintering/melting (SLS/SLM) of aluminium alloy powders: Processing, microstructure, and properties. Progress in Materials Science, 74. 401 - 477. ISSN 0079-6425 https://doi.org/10.1016/j.pmatsci.2015.03.002 © 2015, Elsevier. Licensed under the Creative Commons Attribution-NonCommercial-NoDerivatives 4.0 International http://creativecommons.org/licenses/by-nc-nd/4.0/ Reuse Unless indicated otherwise, fulltext items are protected by copyright with all rights reserved. The copyright exception in section 29 of the Copyright, Designs and Patents Act 1988 allows the making of a single copy solely for the purpose of non-commercial research or private study within the limits of fair dealing. The publisher or other rights-holder may allow further reproduction and re-use of this version - refer to the White Rose Research Online record for this item. Where records identify the publisher as the copyright holder, users can verify any specific terms of use on the publisher’s website. Takedown If you consider content in White Rose Research Online to be in breach of UK law, please notify us by emailing [email protected] including the URL of the record and the reason for the withdrawal request. [email protected] https://eprints.whiterose.ac.uk/ Number of manuscript folios: One hundred and ninety-two (192) pages. -
The Origins of High-Entropy Alloy Contamination Induced by Mechanical Alloying and Sintering
metals Article The Origins of High-Entropy Alloy Contamination Induced by Mechanical Alloying and Sintering Igor Moravcik 1,* , Antonin Kubicek 1, Larissa Moravcikova-Gouvea 1 , Ondrej Adam 1 , Vaclav Kana 2, Vaclav Pouchly 3, Antonin Zadera 2 and Ivo Dlouhy 1 1 Institute of Materials Science and Engineering, Brno University of Technology, Technicka 2896/2, 61669 Brno, Czech Republic; [email protected] (A.K.); [email protected] (L.M.-G.); [email protected] (O.A.); [email protected] (I.D.) 2 Institute of Manufacturing Technology, Brno University of Technology, Technicka 2896/2, 61669 Brno, Czech Republic; [email protected] (V.K.); [email protected] (A.Z.) 3 CEITEC BUT, Brno University of Technology, Purkynova 123, 612 00 Brno, Czech Republic; [email protected] * Correspondence: [email protected]; Tel.: +42-911-566-030 Received: 6 August 2020; Accepted: 28 August 2020; Published: 3 September 2020 Abstract: One of the prevailing problems for materials produced by powder metallurgy is contamination from various sources. This work deals with the influence of process parameters and presence of process control agents (PCA) on the contamination level of materials produced by means of mechanical alloying (MA) technology, densified with spark plasma sintering (SPS). The equiatomic CoCrFeNi high-entropy alloy (HEA) was manufactured by the said methodology. For clear comparison, the 316L austenitic steel powder was milled and densified with identical conditions as a reference material. Both materials were milled in argon and nitrogen atmospheres for various times from 5 to 30 h. Chemical analysis of contamination by carbon, oxygen, and nitrogen within the powder and bulk materials was carried out using combustion analyzers. -
Powder Application in Additive Manufacturing of Metallic Parts Metallic Parts
ProvisionalChapter chapter 8 Powder Application in Additive Manufacturing of Powder Application in Additive Manufacturing of Metallic Parts Metallic Parts Jan Džugan and Zbyšek Nový Jan Džugan and Zbyšek Nový Additional information is available at the end of the chapter Additional information is available at the end of the chapter http://dx.doi.org/10.5772/66874 Abstract This chapter is going to give up-to-date overview of development in the field of addi- tive manufacturing (AM) of metallic components. There will be briefly mentioned input materials and specific requirement for the input materials (powders and wires). General technology process overview will be presented here, and selective laser melting (SLM) technology and beam melting technologies will be described. Advantages of 3D printing technology will be explained in terms of special designs; special properties and gener- ally multifunctional components of production possibilities will be shown. Postprinting procedures leading to improvement of mechanical properties of printed components like thermal or thermomechanical treatment will also be mentioned here. Keywords: additive manufacturing, 3D printing, laser sintering, beam sintering 1. Introduction Additive manufacturing (AM), sometimes called as 3D printing, is a process that is used to manufacture complex 3D products. In additive manufacturing, an object is created by deposi- tion of several layers over each other by computer-controlled deposition process. The objects produced can be of virtually any shape. The components are created by additive manufactur- ing techniques on the basis of computer 3D models. Great attention has been given to this subject recently since it offers new opportunities in factories of the future. -
Pathways to Colonization David V
Pathways To Colonization David V. Smitherman, Jr. NASA, Marshall Space F’light Center, Mail CoLFDO2, Huntsville, AL 35812,256-961-7585, Abstract. The steps required for space colonization are many to grow fiom our current 3-person International Space Station,now under construction, to an inhstmcture that can support hundreds and eventually thousands of people in space. This paper will summarize the author’s fmdings fiom numerous studies and workshops on related subjects and identify some of the critical next steps toward space colonization. Findings will be drawn from the author’s previous work on space colony design, space infirastructure workshops, and various studies that addressed space policy. In cmclusion, this paper will note that siBnifcant progress has been made on space facility construction through the International Space Station program, and that si&icant efforts are needed in the development of new reusable Earth to Orbit transportation systems. The next key steps will include reusable in space transportation systems supported by in space propellant depots, the continued development of inflatable habitat and space elevator technologies, and the resolution of policy issues that will establish a future vision for space development A PATH TO SPACE COLONIZATION In 1993, as part of the author’s duties as a space program planner at the NASA Marshall Space Flight Center, a lengthy timeline was begun to determine the approximate length of time it might take for humans to eventually leave this solar system and travel to the stars. The thought was that we would soon discover a blue planet around another star and would eventually seek to send a colony to explore and expand our presence in this galaxy. -
Additive Manufacturing and Powder Metallurgy. Optimal Product Quality with Tailored Gas Solutions
Additive manufacturing and powder metallurgy. Optimal product quality with tailored gas solutions. Courtesy of TWI 02 Powder metallurgy and additive manufacturing Linde – a world-class gas business. The Linde Group is a world-leading supplier of industrial, process and speciality gases and is one of the most successful global engineering companies. Linde products, gas solutions and services can be found in nearly every industry, in more than 100 countries. Wherever you want to go – we are there to help. Linde worldwide Powder metallurgy and additive manufacturing 03 Contents. 2 Linde – a world-class gas business 17 Heat treatment and surface cleaning 18 Sintering 5 Overview of additive manufacturing 19 Hot isostatic pressing and powder metallurgy processes 20 Cleaning 7 Metal powder production 21 Linde – leading in powder metallurgy and additive manufacturing innovation 9 Additive manufacturing processes 22 Linde – world class gas supply options 10 Laser metal fusion 11 Laser metal deposition 12 Selective laser sintering 23 Linde – the leading gas partner 13 Wire arc additive manufacturing 14 Thermal spraying 15 Electron beam melting 04 Powder metallurgy and additive manufacturing Courtesy of TWI Powder metallurgy and additive manufacturing 05 Overview of additive manufacturing and powder metallurgy processes. The metal powder industry has grown significantly in recent years – Metal powder production due to the development and use of sintered parts and additive – Additive manufacturing processes: manufacturing processes. Linde is at the forefront of research and – Laser metal fusion (LMF) development not just for the metal powder industry and the metal – Laser metal deposition (LMD) additive manufacturing industry, but also for the entire powder value – Selective laser sintering (SLS) chain in additive manufacturing. -
Tung Sten Properties, Chemistry, Technology of the Element, Alloys, and Chemical Compounds
Tung sten Properties, Chemistry, Technology of the Element, Alloys, and Chemical Compounds Erik Lassner and Wolf-Dieter Schubert nn Unvrt f hnl nn, Atr Kluwer Academie / Plenum Publishers New York, Boston, Dordrecht, London, Moscow Contents CHAPTER 1. The Element Tungsten: Its Properties 1.1. Analogous to Atom Related Physical Properties 1 1.1.1. Nucleus 2 1.1.2. Electron Configuration 2 1.1.3. Spectra 3 1.1.4. Thermodynamic Functions 6 1.2. Bulk Tungsten Metal Related Physical Properties 7 1.2.1. Electronic Structure and Bonding 7 1.2.2. Structural Properties 11 1.2.3. Mechanical Properties 16 1.2.4. Thermal Properties 30 1.2.5. Electromagnetic Properties 34 1.2.6. Optical Properties 36 1.2.7. Electron Emission 40 1.2.8. Acoustic Properties 42 1.3. Chemical Properties of Tungsten Metal 42 1.3.1. General Remarks 42 1.3.2. Reactions with Nonmetals 46 1.3.3. Reactions with Metals 46 1.3.4. Reactions with Compounds 50 1.3.5. Reactions with Aqueous Solutions 53 1.3.6. Miscellaneous 55 1.3.7. Reactions with Organic Compounds 56 References for Chapter 1 56 CHAPTER 2. Tungsten History: From Genesis to the 20th Century Products 2.1. The Formation of Tungsten Atoms 61 2.2 How Tungsten Atoms Came on Earth 63 2.3. Average Abundance 64 2.4. Geology: Formation of Ore Deposits 65 Ulf xv COES 2.5. Minerals 69 2.6. Ore Deposits and Reserves 70 2.7. Early Discoveries of Ores, Compounds, and of the Element 77 2.8. -
A New Hope for International Space
View metadata, citation and similar papers at core.ac.uk brought to you by CORE provided by Seton Hall University Libraries A NEW HOPE FOR INTERNATIONAL SPACE LAW: INCORPORATING NINETEENTH CENTURY FIRST POSSESSION PRINCIPLES INTO THE 1967 SPACE TREATY FOR THE COLONIZATION OF OUTER SPACE IN THE TWENTY-FIRST CENTURY Brandon C. Gruner∗ INTRODUCTION Legend has it that a friend once asked Mark Twain what he should invest in, and the good-humored author responded “Buy land; they’ve stopped making it.”1 The author’s advice was given more than 100 years ago and it still makes good sense. If a resource is scarce, it is almost always going to become more valuable.2 It is ∗ J.D. Candidate, 2005, Seton Hall University School of Law; B.A. Magna Cum Laude, Phi Beta Kappa, History, 1999, New York University: College of Arts and Science. I would like to thank my parents, Robert and Maria Gruner, for their unwavering support throughout my education, and Paula A. Franzese, the Peter W. Rodino Professor of Law at Seton Hall Law School, for her invaluable knowledge and guidance in writing this Comment. 1 A search of the Lexis and Westlaw databases, as well as the World Wide Web, demonstrates that hundreds of newspapers and Web sites have attributed this quote or some similar version to Mark Twain. See, e.g., Clinton J. Fynes, “Ambassadorial Role” Leads Ian to Property, COURIER-MAIL, Oct. 12, 1990 (relaying the story of Mark Twain advising his friend to “Buy land, they’re not making any more of it.”); Tom Fegely, Lack of Action on Tag Fees Pinches Gamelands Purchases, Maintenance, MORNING CALL (Allentown, PA), Sept. -
Colonization of Venus
Conference on Human Space Exploration, Space Technology & Applications International Forum, Albuquerque, NM, Feb. 2-6 2003. Colonization of Venus Geoffrey A. Landis NASA Glenn Research Center mailstop 302-1 21000 Brook Park Road Cleveland, OH 44135 21 6-433-2238 geofrq.landis@grc. nasa.gov ABSTRACT Although the surface of Venus is an extremely hostile environment, at about 50 kilometers above the surface the atmosphere of Venus is the most earthlike environment (other than Earth itself) in the solar system. It is proposed here that in the near term, human exploration of Venus could take place from aerostat vehicles in the atmosphere, and that in the long term, permanent settlements could be made in the form of cities designed to float at about fifty kilometer altitude in the atmosphere of Venus. INTRODUCTION Since Gerard K. O'Neill [1974, 19761 first did a detailed analysis of the concept of a self-sufficient space colony, the concept of a human colony that is not located on the surface of a planet has been a major topic of discussion in the space community. There are many possible economic justifications for such a space colony, including use as living quarters for a factory producing industrial products (such as solar power satellites) in space, and as a staging point for asteroid mining [Lewis 19971. However, while the concept has focussed on the idea of colonies in free space, there are several disadvantages in colonizing empty space. Space is short on most of the raw materials needed to sustain human life, and most particularly in the elements oxygen, hydrogen, carbon, and nitrogen. -
Space Resources : Social Concerns / Editors, Mary Fae Mckay, David S
Frontispiece Advanced Lunar Base In this panorama of an advanced lunar base, the main habitation modules in the background to the right are shown being covered by lunar soil for radiation protection. The modules on the far right are reactors in which lunar soil is being processed to provide oxygen. Each reactor is heated by a solar mirror. The vehicle near them is collecting liquid oxygen from the reactor complex and will transport it to the launch pad in the background, where a tanker is just lifting off. The mining pits are shown just behind the foreground figure on the left. The geologists in the foreground are looking for richer ores to mine. Artist: Dennis Davidson NASA SP-509, vol. 4 Space Resources Social Concerns Editors Mary Fae McKay, David S. McKay, and Michael B. Duke Lyndon B. Johnson Space Center Houston, Texas 1992 National Aeronautics and Space Administration Scientific and Technical Information Program Washington, DC 1992 For sale by the U.S. Government Printing Office Superintendent of Documents, Mail Stop: SSOP, Washington, DC 20402-9328 ISBN 0-16-038062-6 Technical papers derived from a NASA-ASEE summer study held at the California Space Institute in 1984. Library of Congress Cataloging-in-Publication Data Space resources : social concerns / editors, Mary Fae McKay, David S. McKay, and Michael B. Duke. xii, 302 p. : ill. ; 28 cm.—(NASA SP ; 509 : vol. 4) 1. Outer space—Exploration—United States. 2. Natural resources. 3. Space industrialization—United States. I. McKay, Mary Fae. II. McKay, David S. III. Duke, Michael B. IV. United States. -
Risks of Space Colonization
Risks of space colonization Marko Kovic∗ July 2020 Abstract Space colonization is humankind's best bet for long-term survival. This makes the expected moral value of space colonization immense. However, colonizing space also creates risks | risks whose potential harm could easily overshadow all the benefits of humankind's long- term future. In this article, I present a preliminary overview of some major risks of space colonization: Prioritization risks, aberration risks, and conflict risks. Each of these risk types contains risks that can create enormous disvalue; in some cases orders of magnitude more disvalue than all the potential positive value humankind could have. From a (weakly) negative, suffering-focused utilitarian view, we there- fore have the obligation to mitigate space colonization-related risks and make space colonization as safe as possible. In order to do so, we need to start working on real-world space colonization governance. Given the near total lack of progress in the domain of space gover- nance in recent decades, however, it is uncertain whether meaningful space colonization governance can be established in the near future, and before it is too late. ∗[email protected] 1 1 Introduction: The value of colonizing space Space colonization, the establishment of permanent human habitats beyond Earth, has been the object of both popular speculation and scientific inquiry for decades. The idea of space colonization has an almost poetic quality: Space is the next great frontier, the next great leap for humankind, that we hope to eventually conquer through our force of will and our ingenuity. From a more prosaic point of view, space colonization is important because it represents a long-term survival strategy for humankind1.