An Homeotic Post-Transcriptional Network Controlled by the RNA
Total Page:16
File Type:pdf, Size:1020Kb
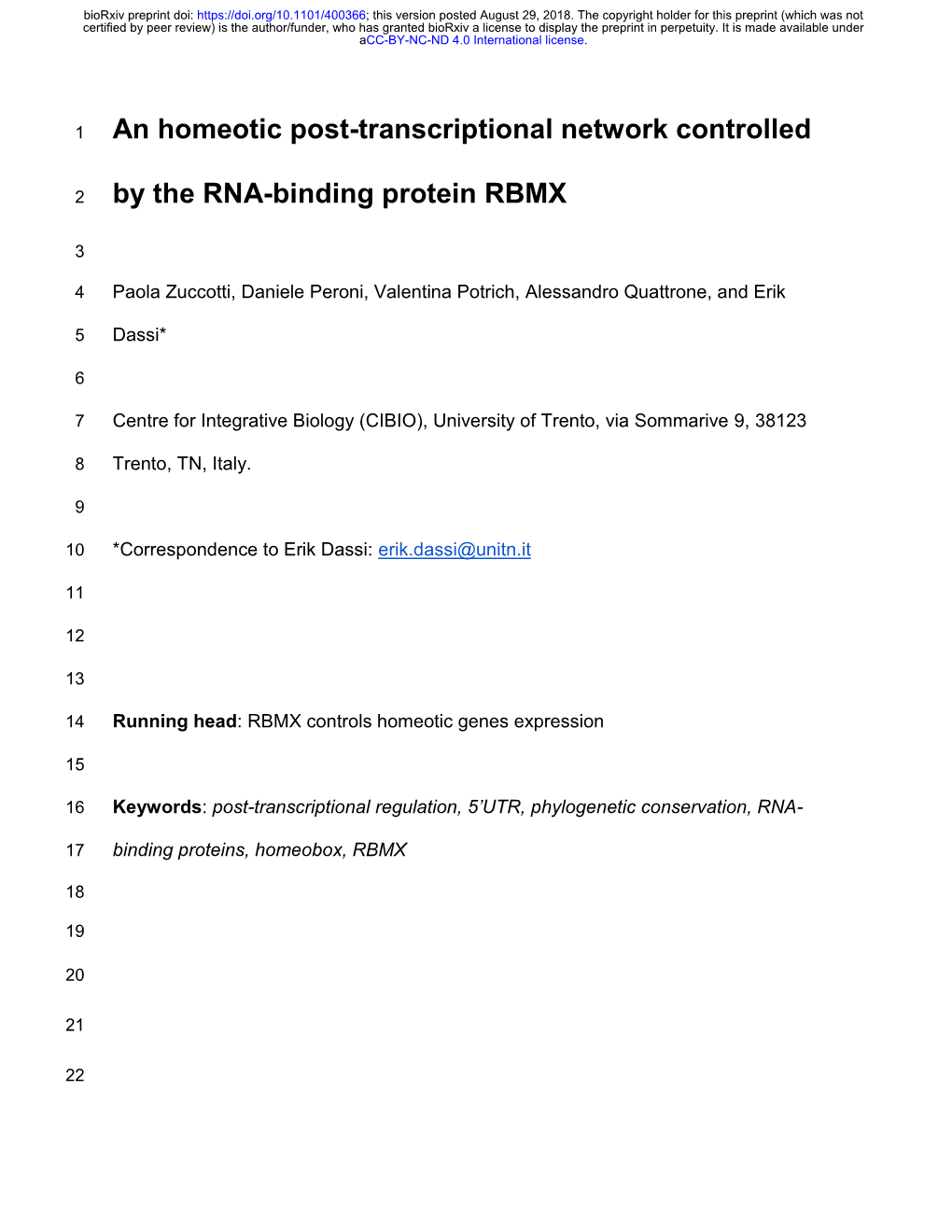
Load more
Recommended publications
-
A Computational Approach for Defining a Signature of Β-Cell Golgi Stress in Diabetes Mellitus
Page 1 of 781 Diabetes A Computational Approach for Defining a Signature of β-Cell Golgi Stress in Diabetes Mellitus Robert N. Bone1,6,7, Olufunmilola Oyebamiji2, Sayali Talware2, Sharmila Selvaraj2, Preethi Krishnan3,6, Farooq Syed1,6,7, Huanmei Wu2, Carmella Evans-Molina 1,3,4,5,6,7,8* Departments of 1Pediatrics, 3Medicine, 4Anatomy, Cell Biology & Physiology, 5Biochemistry & Molecular Biology, the 6Center for Diabetes & Metabolic Diseases, and the 7Herman B. Wells Center for Pediatric Research, Indiana University School of Medicine, Indianapolis, IN 46202; 2Department of BioHealth Informatics, Indiana University-Purdue University Indianapolis, Indianapolis, IN, 46202; 8Roudebush VA Medical Center, Indianapolis, IN 46202. *Corresponding Author(s): Carmella Evans-Molina, MD, PhD ([email protected]) Indiana University School of Medicine, 635 Barnhill Drive, MS 2031A, Indianapolis, IN 46202, Telephone: (317) 274-4145, Fax (317) 274-4107 Running Title: Golgi Stress Response in Diabetes Word Count: 4358 Number of Figures: 6 Keywords: Golgi apparatus stress, Islets, β cell, Type 1 diabetes, Type 2 diabetes 1 Diabetes Publish Ahead of Print, published online August 20, 2020 Diabetes Page 2 of 781 ABSTRACT The Golgi apparatus (GA) is an important site of insulin processing and granule maturation, but whether GA organelle dysfunction and GA stress are present in the diabetic β-cell has not been tested. We utilized an informatics-based approach to develop a transcriptional signature of β-cell GA stress using existing RNA sequencing and microarray datasets generated using human islets from donors with diabetes and islets where type 1(T1D) and type 2 diabetes (T2D) had been modeled ex vivo. To narrow our results to GA-specific genes, we applied a filter set of 1,030 genes accepted as GA associated. -
High-Throughput Discovery of Novel Developmental Phenotypes
High-throughput discovery of novel developmental phenotypes The Harvard community has made this article openly available. Please share how this access benefits you. Your story matters Citation Dickinson, M. E., A. M. Flenniken, X. Ji, L. Teboul, M. D. Wong, J. K. White, T. F. Meehan, et al. 2016. “High-throughput discovery of novel developmental phenotypes.” Nature 537 (7621): 508-514. doi:10.1038/nature19356. http://dx.doi.org/10.1038/nature19356. Published Version doi:10.1038/nature19356 Citable link http://nrs.harvard.edu/urn-3:HUL.InstRepos:32071918 Terms of Use This article was downloaded from Harvard University’s DASH repository, and is made available under the terms and conditions applicable to Other Posted Material, as set forth at http:// nrs.harvard.edu/urn-3:HUL.InstRepos:dash.current.terms-of- use#LAA HHS Public Access Author manuscript Author ManuscriptAuthor Manuscript Author Nature. Manuscript Author Author manuscript; Manuscript Author available in PMC 2017 March 14. Published in final edited form as: Nature. 2016 September 22; 537(7621): 508–514. doi:10.1038/nature19356. High-throughput discovery of novel developmental phenotypes A full list of authors and affiliations appears at the end of the article. Abstract Approximately one third of all mammalian genes are essential for life. Phenotypes resulting from mouse knockouts of these genes have provided tremendous insight into gene function and congenital disorders. As part of the International Mouse Phenotyping Consortium effort to generate and phenotypically characterize 5000 knockout mouse lines, we have identified 410 Users may view, print, copy, and download text and data-mine the content in such documents, for the purposes of academic research, subject always to the full Conditions of use:http://www.nature.com/authors/editorial_policies/license.html#terms #Corresponding author: [email protected]. -
RBMX Is a Novel Hepatic Transcriptional Regulator of SREBP-1C Gene Response to High-Fructose Diet
View metadata, citation and similar papers at core.ac.uk brought to you by CORE provided by Elsevier - Publisher Connector FEBS Letters 581 (2007) 218–222 RBMX is a novel hepatic transcriptional regulator of SREBP-1c gene response to high-fructose diet Tadashi Takemotoa, Yoshihiko Nishiob,*, Osamu Sekineb, Chikako Ikeuchib, Yoshio Nagaib, Yasuhiro Maenob, Hiroshi Maegawab, Hiroshi Kimuraa, Atsunori Kashiwagib a Division of Molecular Genetics in Medicine, Department of Biochemistry and Molecular Biology, Shiga University of Medical Science, Shiga 520-2192, Japan b Division of Endocrinology and Metabolism, Department of Medicine, Shiga University of Medical Science, Shiga 520-2192, Japan Received 24 October 2006; accepted 1 December 2006 Available online 14 December 2006 Edited by Berend Wieringa nisms by which the high-fructose diet induces SREBP-1c gene Abstract In rodents a high-fructose diet induces metabolic derangements similar to those in metabolic syndrome. Previously expression in the liver. we suggested that in mouse liver an unidentified nuclear protein We previously reported a variation of susceptibility to these binding to the sterol regulatory element (SRE)-binding protein- metabolic disorders after monitoring the effects of high-fruc- 1c (SREBP-1c) promoter region plays a key role for the re- tose diet in different mouse strains [6]. Ten strains of inbred sponse to high-fructose diet. Here, using MALDI-TOF MASS mice were separated into CBA and DBA groups according technique, we identified an X-chromosome-linked RNA binding to their response to high-fructose diet. The hepatic mRNA motif protein (RBMX) as a new candidate molecule. In electro- expression of SREBP-1c in CBA/JN mice was remarkably ele- phoretic mobility shift assay, anti-RBMX antibody displaced the vated by high-fructose diet but not in DBA/2N. -
An Ancient Germ Cell-Specific RNA-Binding Protein Protects
RESEARCH ARTICLE An ancient germ cell-specific RNA-binding protein protects the germline from cryptic splice site poisoning Ingrid Ehrmann1, James H Crichton2, Matthew R Gazzara3,4, Katherine James5, Yilei Liu1,6, Sushma Nagaraja Grellscheid1,7, TomazˇCurk8, Dirk de Rooij9,10, Jannetta S Steyn11, Simon Cockell11, Ian R Adams2, Yoseph Barash3,12*, David J Elliott1* 1Institute of Genetic Medicine, Newcastle University, Newcastle, United Kingdom; 2MRC Human Genetics Unit, MRC Institute of Genetics and Molecular Medicine, University of Edinburgh, Edinburgh, United Kingdom; 3Department of Genetics, Perelman School of Medicine, University of Pennsylvania, Philadelphia, United States; 4Department of Biochemistry and Biophysics, Perelman School of Medicine, University of Pennsylvania, Philadelphia, United States; 5Life Sciences, Natural History Museum, London, United Kingdom; 6Department of Plant and Microbial Biology, University of Zu¨ rich, Zu¨ rich, Switzerland; 7School of Biological and Biomedical Sciences, University of Durham, Durham, United Kingdom; 8Laboratory of Bioinformatics, Faculty of Computer and Information Sciences, University of Ljubljana, Ljubljana, Slovenia; 9Reproductive Biology Group, Division of Developmental Biology, Department of Biology, Faculty of Science, Utrecht University, Utrecht, The Netherlands; 10Center for Reproductive Medicine, Academic Medical Center, University of Amsterdam, Amsterdam, The Netherlands; 11Bioinformatics Support Unit, Faculty of Medical Sciences, Newcastle University, Newcastle, United Kingdom; 12Department of Computer and Information Science, University of Pennsylvania, Philadelphia, United States *For correspondence: [email protected] (YB); [email protected] (DJE) Competing interests: The Abstract Male germ cells of all placental mammals express an ancient nuclear RNA binding authors declare that no protein of unknown function called RBMXL2. Here we find that deletion of the retrogene encoding competing interests exist. -
The X-Chromosome RBMX Gene Is Expressed in Mammary Carcinoma
CANCER GENOMICS & PROTEOMICS 1: 39-44 (2004) The X-chromosome RBMX Gene is Expressed in Mammary Carcinoma F. GÓMEZ-ESQUERl, D. AGUDOl , F. MARTÍNEZ-ARRIBAS2, M.J. NUNEZ-VILLAR2, M. POLLÁN3 and J. SCHNEIDERl ,2 lUniversidad Rey Juan Carlos, Facultad de Ciencias de la Salud, Madrid; 2Fundación Tejerina-Centro de Patología de la Mama, Madrid; 3Centro Nacional de Epidemiologta, Madrid, Spain Abstract. Background: A gene located on the qll.23 regíon of conserved gender-specific genes, such as the ones regulating the the male chromosome, RBMY, which plays a role in male phenotype and spermatogenesis (3). The X chromosome, spermatogenesis, is down-regulated in testicular cancer. RBMY is in its turn, undergoes a specific phenomenon in females, known a diverged X-Y shared gene. The corresponding X chromosome as "X--chromosome inactivation", by which one of both alleles is gene, RBMX, is located on Xq26. Materials and Methods: We switched off at a very early stage of the embryonic development, studied fresh tissues from 122 infiltrating breast cancel':>' (99 ductal in order to avoid a double expression of genes if compared to infiltrating, 1910bular infiltrating and 4 tubular carcinomas) for their male counterparts. Sinee the inactivation of one or the the expression ofRBMXby means ofdífferential RT-PCR (reverse other allele occurs in a random fashion, adult females in the transcription-polymerase chain reaction), using beta-actin as an end are a mosaie of cells with either X allele inactivated (4). internal control and normalization standard, The obtained results The outcome of this regulatory proeess is that most genes of were compared with all available clinical and molecular data of the X chromosome are transcriptionally silenced, although the studied tumors (estrogen and progesterone receptors (ER & alterations do occur and are generally associated with grave PR), c-erb-B2, p53, Ki67, DNA-ploidy, Bcl-2, VEGF, CD105 disorders,like the Wiskott-Aldrich, the Leseh-Nyhan or the (endoglin), histologic variety, histologic and nuclear grade and Barth syndromes. -
RBMX Family Proteins Connect the Fields of Nuclear RNA Processing
International Journal of Biochemistry and Cell Biology 108 (2019) 1–6 Contents lists available at ScienceDirect International Journal of Biochemistry and Cell Biology journal homepage: www.elsevier.com/locate/biocel RBMX family proteins connect the fields of nuclear RNA processing, disease and sex chromosome biology T ⁎ David J. Elliott , Caroline Dalgliesh, Gerald Hysenaj, Ingrid Ehrmann Institute of Genetic Medicine, Newcastle University, Newcastle-upon-Tyne, NE1 3BZ, UK ARTICLE INFO ABSTRACT Keywords: RBMX is a ubiquitously expressed nuclear RNA binding protein that is encoded by a gene on the X chromosome. RNA splicing RBMX belongs to a small protein family with additional members encoded by paralogs on the mammalian Y Gene expression chromosome and other chromosomes. These RNA binding proteins are important for normal development, and Brain development also implicated in cancer and viral infection. At the molecular level RBMX family proteins contribute to splicing Testis control, transcription and genome integrity. Establishing what endogenous genes and pathways are controlled by Cancer RBMX and its paralogs will have important implications for understanding chromosome biology, DNA repair and mammalian development. Here we review what is known about this family of RNA binding proteins, and identify important current questions about their functions. 1. Introduction et al., 1993). While RBMX is expressed ubiquitously through the body, RBMY genes are specifically expressed in germ cells (cells that even- RBMX (an acronym of RNA Binding Motif protein, X-linked) was tually develop into sperm). Multiple RBMY genes are present on the originally identified as one of a functionally diverse group of nuclear long arm of the human Y chromosome, each encoding 496 amino acid proteins that bind to polyadenylated RNA. -
Rare Cnvs Provide Novel Insights Into the Molecular Basis of GH and IGF-1 Insensitivity
6 183 E Cottrell and others CNVs in GH and IGF-1 183:6 581–595 Clinical Study insensitivity Rare CNVs provide novel insights into the molecular basis of GH and IGF-1 insensitivity Emily Cottrell1, Claudia P Cabrera2,3, Miho Ishida4, Sumana Chatterjee1, James Greening5, Neil Wright6, Artur Bossowski7, Leo Dunkel1, Asma Deeb8, Iman Al Basiri9, Stephen J Rose10, Avril Mason11, Susan Bint12, Joo Wook Ahn13, Vivian Hwa14, Louise A Metherell1, Gudrun E Moore4 and Helen L Storr1 1Centre for Endocrinology, William Harvey Research Institute, Barts and the London School of Medicine & Dentistry, Queen Mary University of London, London, UK, 2Centre for Translational Bioinformatics, Queen Mary University of London, London, UK, 3NIHR Barts Cardiovascular Biomedical Research Centre, Barts and The London School of Medicine and Dentistry, Queen Mary University of London, London, UK, 4University College London, Great Ormond Street Institute of Child Health, London, UK, 5University Hospitals of Leicester NHS Trust, Leicester, UK, 6The University of Sheffield Faculty of Medicine, Dentistry and Health, Sheffield, UK, 7Department of Pediatrics, Endocrinology and Diabetes with a Cardiology Unit, Medical University of Bialystok, Bialystok, Poland, 8Paediatric Endocrinology Department, Mafraq Hospital, Abu Dhabi, United Arab Emirates, 9Mubarak Al-kabeer Hospital, Jabriya, Kuwait, 10University Hospitals Birmingham NHS Foundation Trust, Birmingham, UK, 11Royal Correspondence Hospital for Children, Glasgow, UK, 12Viapath, Guy’s Hospital, London, UK, 13Addenbrookes Hospital, Cambridge, UK, should be addressed and 14Cincinnati Center for Growth Disorders, Division of Endocrinology, Cincinnati Children’s Hospital Medical to H L Storr Center, Department of Pediatrics, University of Cincinnati College of Medicine, Cincinnati, Ohio, USA Email [email protected] Abstract Objective: Copy number variation (CNV) has been associated with idiopathic short stature, small for gestational age and Silver-Russell syndrome (SRS). -
Evolutionary Strata on the Mouse X Chromosome Correspond to Strata on the Human X Chromosome Sara A
Downloaded from genome.cshlp.org on September 30, 2021 - Published by Cold Spring Harbor Laboratory Press Letter Evolutionary Strata on the Mouse X Chromosome Correspond to Strata on the Human X Chromosome Sara A. Sandstedt1 and Priscilla K. Tucker Department of Ecology and Evolutionary Biology, and Museum of Zoology, University of Michigan, Ann Arbor, Michigan 48109, USA Lahn and Page previously observed that genes on the human X chromosome were physically arranged along the chromosome in “strata,” roughly ordered by degree ofdivergence fromrela ted genes on the Y chromosome. They hypothesized that this ordering results from a historical series of suppressions ofrecombination along the mammalian Y chromosome, thereby allowing formerly recombining X and Y chromosomal genes to diverge independently. Here predictions ofthis hypothesis are confirmedin a non primate mammalian order, Rodentia, through an analysis ofeight gene pairs fromthe X and Y chromosomes ofthe ho use mouse, Mus musculus. The mouse X chromosome has been rearranged relative to the human X, so strata were not found in the same physical order on the mouse X. However, based on synonymous evolutionary distances, X-linked genes in M. musculus fall into the same strata as orthologous genes in humans, as predicted. The boundary between strata 2 and 3 is statistically significant, but the boundary between strata 1 and 2 is not significant in mice. An analysis ofsmaller fragmentsofSmcy, Smcx, Zfy, and Zfx from seven species of Mus confirmed that the strata in Mus musculus were representative ofthe genus Mus. [Supplemental material is available online at www.genome.org. The sequence data from this study have been submitted to GenBank under accession nos. -
PARP-1–Dependent Recruitment of Cold-Inducible RNA-Binding Protein
PARP-1–dependent recruitment of cold-inducible PNAS PLUS RNA-binding protein promotes double-strand break repair and genome stability Jung-Kuei Chena, Wen-Ling Lina, Zhang Chenb, and Hung-wen Liua,b,c,1 aInstitute for Cellular and Molecular Biology, The University of Texas at Austin, Austin, TX 78712; bDepartment of Chemistry, The University of Texas at Austin, Austin, TX 78712; and cDivision of Chemical Biology and Medicinal Chemistry, College of Pharmacy, The University of Texas at Austin, Austin, TX 78712 Edited by Richard D. Kolodner, Ludwig Institute for Cancer Research, La Jolla, CA, and approved January 12, 2018 (received for review August 12, 2017) Maintenance of genome integrity is critical for both faithful propa- modify itself and other protein targets during the early stages of gation of genetic information and prevention of mutagenesis in- DNA damage responses (DDRs), such as local chromatin re- duced by various DNA damage events. Here we report cold-inducible laxation, transcription regulation, and recruitment of DNA repair RNA-binding protein (CIRBP) as a newly identified key regulator machinery (11, 12). Emerging evidence indicates that PARP-1 and in DNA double-strand break (DSB) repair. On DNA damage, CIRBP PAR polymer play active roles in the localization of RNA-binding temporarily accumulates at the damaged regions and is poly(ADP proteins at the site of DNA damage (13–18). In particular, PARP-1 ribosyl)ated by poly(ADP ribose) polymerase-1 (PARP-1). Its dissoci- recruits FUS protein to damaged chromatin, which is a prerequisite ation from the sites of damage may depend on its phosphorylation for both HR and NHEJ pathways (15, 16). -
RBMX Enables Productive RNA Processing of Ultra-Long Exons
bioRxiv preprint doi: https://doi.org/10.1101/2020.10.09.333039; this version posted October 9, 2020. The copyright holder for this preprint (which was not certified by peer review) is the author/funder, who has granted bioRxiv a license to display the preprint in perpetuity. It is made available under aCC-BY 4.0 International license. 1 RBMX enables productive RNA processing of ultra-long exons important for 2 genome stability 3 Sara Luzzi1*, Gerald Hysenaj1*, Chileleko Siachisumo1*, Kathleen Cheung2, Matthew 4 Gazzara3,4, Katherine James5, Caroline Dalgliesh1, Mahsa Kheirollahi Chadegani1, 5 Ingrid Ehrmann1, Graham R Smith2, Simon J Cockell2, Jennifer Munkley1, Yoseph 6 Barash3,6 and David J Elliott1†. 7 8 1 Biosciences Institute, Faculty of Medical Sciences, Newcastle University, Newcastle, 9 United Kingdom 10 2 Bioinformatics Support Unit, Faculty of Medical Sciences, Newcastle University, Newcastle, 11 United Kingdom. 12 3 Department of Genetics, Perelman School of Medicine, University of Pennsylvania, 13 Philadelphia, United States. 14 4 Department of Biochemistry and Biophysics, Perelman School of Medicine, University of 15 Pennsylvania, Philadelphia, United States. 16 5 Department of Applied Sciences, Northumbria University, Newcastle, United Kingdom 17 6 Department of Computer and Information Science, University of Pennsylvania, 18 Philadelphia, United States 19 20 * These authors contributed equally to the paper as first authors † 21 To whom correspondence should be addressed. Email: [email protected] 1 bioRxiv preprint doi: https://doi.org/10.1101/2020.10.09.333039; this version posted October 9, 2020. The copyright holder for this preprint (which was not certified by peer review) is the author/funder, who has granted bioRxiv a license to display the preprint in perpetuity. -
PUMILIO, but Not RBMX, Binding Is Required for Regulation of Genomic
RESEARCH ADVANCE PUMILIO, but not RBMX, binding is required for regulation of genomic stability by noncoding RNA NORAD Mahmoud M Elguindy1,2, Florian Kopp1, Mohammad Goodarzi3, Frederick Rehfeld1, Anu Thomas1, Tsung-Cheng Chang1, Joshua T Mendell1,4,5,6* 1Department of Molecular Biology, University of Texas Southwestern Medical Center, Dallas, United States; 2Medical Scientist Training Program, University of Texas Southwestern Medical Center, Dallas, United States; 3Department of Biochemistry, University of Texas Southwestern Medical Center, Dallas, United States; 4Harold C Simmons Comprehensive Cancer Center, University of Texas Southwestern Medical Center, Dallas, United States; 5Hamon Center for Regenerative Science and Medicine, University of Texas Southwestern Medical Center, Dallas, United States; 6Howard Hughes Medical Institute, University of Texas Southwestern Medical Center, Dallas, United States Abstract NORAD is a conserved long noncoding RNA (lncRNA) that is required for genome stability in mammals. NORAD acts as a negative regulator of PUMILIO (PUM) proteins in the cytoplasm, and we previously showed that loss of NORAD or PUM hyperactivity results in genome instability and premature aging in mice (Kopp et al., 2019). Recently, however, it was reported that NORAD regulates genome stability through an interaction with the RNA binding protein RBMX in the nucleus. Here, we addressed the contributions of NORAD:PUM and NORAD:RBMX interactions to genome maintenance by this lncRNA in human cells. Extensive RNA FISH and fractionation experiments established that NORAD localizes predominantly to the cytoplasm with or without *For correspondence: DNA damage. Moreover, genetic rescue experiments demonstrated that PUM binding is required joshua.mendell@utsouthwestern. for maintenance of genomic stability by NORAD whereas binding of RBMX is dispensable for this edu function. -
Us 2018 / 0305689 A1
US 20180305689A1 ( 19 ) United States (12 ) Patent Application Publication ( 10) Pub . No. : US 2018 /0305689 A1 Sætrom et al. ( 43 ) Pub . Date: Oct. 25 , 2018 ( 54 ) SARNA COMPOSITIONS AND METHODS OF plication No . 62 /150 , 895 , filed on Apr. 22 , 2015 , USE provisional application No . 62/ 150 ,904 , filed on Apr. 22 , 2015 , provisional application No. 62 / 150 , 908 , (71 ) Applicant: MINA THERAPEUTICS LIMITED , filed on Apr. 22 , 2015 , provisional application No. LONDON (GB ) 62 / 150 , 900 , filed on Apr. 22 , 2015 . (72 ) Inventors : Pål Sætrom , Trondheim (NO ) ; Endre Publication Classification Bakken Stovner , Trondheim (NO ) (51 ) Int . CI. C12N 15 / 113 (2006 .01 ) (21 ) Appl. No. : 15 /568 , 046 (52 ) U . S . CI. (22 ) PCT Filed : Apr. 21 , 2016 CPC .. .. .. C12N 15 / 113 ( 2013 .01 ) ; C12N 2310 / 34 ( 2013. 01 ) ; C12N 2310 /14 (2013 . 01 ) ; C12N ( 86 ) PCT No .: PCT/ GB2016 /051116 2310 / 11 (2013 .01 ) $ 371 ( c ) ( 1 ) , ( 2 ) Date : Oct . 20 , 2017 (57 ) ABSTRACT The invention relates to oligonucleotides , e . g . , saRNAS Related U . S . Application Data useful in upregulating the expression of a target gene and (60 ) Provisional application No . 62 / 150 ,892 , filed on Apr. therapeutic compositions comprising such oligonucleotides . 22 , 2015 , provisional application No . 62 / 150 ,893 , Methods of using the oligonucleotides and the therapeutic filed on Apr. 22 , 2015 , provisional application No . compositions are also provided . 62 / 150 ,897 , filed on Apr. 22 , 2015 , provisional ap Specification includes a Sequence Listing . SARNA sense strand (Fessenger 3 ' SARNA antisense strand (Guide ) Mathew, Si Target antisense RNA transcript, e . g . NAT Target Coding strand Gene Transcription start site ( T55 ) TY{ { ? ? Targeted Target transcript , e .