Biodegradability of Chlorinated Aromatic Compounds
Total Page:16
File Type:pdf, Size:1020Kb
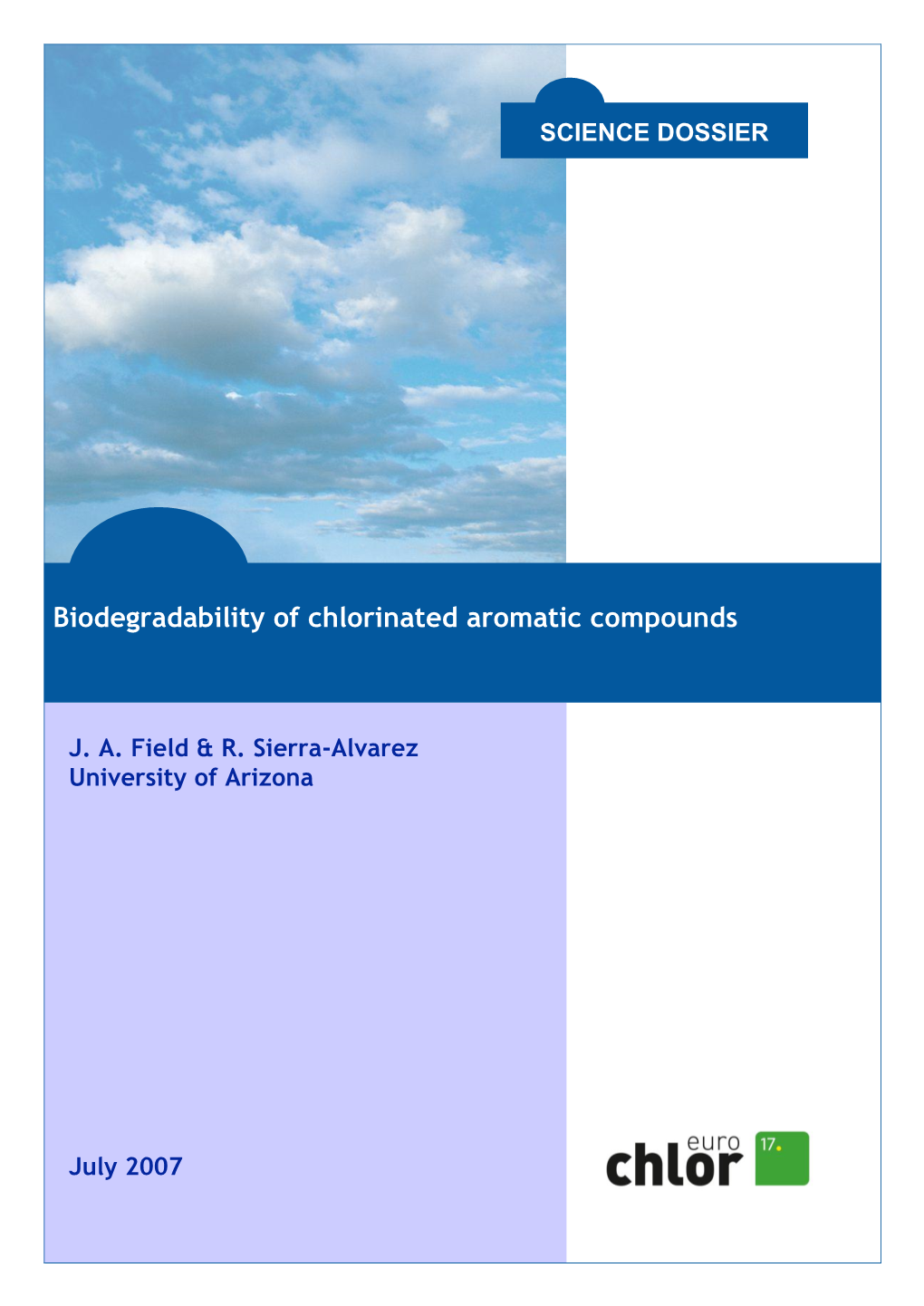
Load more
Recommended publications
-
Panellus Stipticus
VOLUME 55: 5 SEPTEMBER-OCTOBER 2015 www.namyco.org Regional Trustee Nominations Every year, on a rotating basis, four Regional Trustee positions are due for nomination and election by NAMA members in their respective region. The following regions have openings for three-year terms to begin in 2016: Appalachian, Boreal, Great Lakes, and Rocky Mountain. The affiliated clubs for each region are listed below; those without a club affiliation are members of the region where they live. Members of each region may nominate them- selves or another person in that region. Nominations close on October 31, 2015. Appalachian Cumberland Mycological Society Mushroom Club of Georgia North Alabama Mushroom Society South Carolina Upstate Mycological Society West Virginia Mushroom Club Western Pennsylvania Mushroom Club Boreal Alberta Mycological Society Foray Newfoundland & Labrador Great Lakes Hoosier Mushroom Society Illinois Mycological Association Michigan Mushroom Hunters Club Minnesota Mycological Society Mycological Society of Toronto Four Corners Mushroom Club Ohio Mushroom Society Mushroom Society of Utah Wisconsin Mycological Society New Mexico Mycological Society Rocky Mountains North Idaho Mycological Association Arizona Mushroom Club Pikes Peak Mycological Society Colorado Mycological Society Southern Idaho Mycological Association SW Montana Mycological Association Please send the information outlined on the form below to Adele Mehta by email: [email protected], or by mail: 4917 W. Old Shakopee Road, Bloomington, MN 55437. Regional -
Bioluminescence in Mushroom and Its Application Potentials
Nigerian Journal of Science and Environment, Vol. 14 (1) (2016) BIOLUMINESCENCE IN MUSHROOM AND ITS APPLICATION POTENTIALS Ilondu, E. M.* and Okiti, A. A. Department of Botany, Faculty of Science, Delta State University, Abraka, Nigeria. *Corresponding author. E-mail: [email protected]. Tel: 2348036758249. ABSTRACT Bioluminescence is a biological process through which light is produced and emitted by a living organism resulting from a chemical reaction within the body of the organism. The mechanism behind this phenomenon is an oxygen-dependent reaction involving substrates generally termed luciferin, which is catalyzed by one or more of an assortment of unrelated enzyme called luciferases. The history of bioluminescence in fungi can be traced far back to 382 B.C. when it was first noted by Aristotle in his early writings. It is the nature of bioluminescent mushrooms to emit a greenish light at certain stages in their life cycle and this light has a maximum wavelength range of 520-530 nm. Luminescence in mushroom has been hypothesized to attract invertebrates that aids in spore dispersal and testing for pollutants (ions of mercury) in water supply. The metabolites from luminescent mushrooms are effectively bioactive in anti-moulds, anti-bacteria, anti-virus, especially in inhibiting the growth of cancer cell and very useful in areas of biology, biotechnology and medicine as luminescent markers for developing new luminescent microanalysis methods. Luminescent mushroom is a novel area of research in the world which is beneficial to mankind especially with regards to environmental pollution monitoring and biomedical applications. Bioluminescence in fungi is a beautiful phenomenon to observe which should be of interest to Scientists of all endeavors. -
May 2015 Newsletter of the Central New York Mycological Society ______
May 2015 Newsletter of the Central New York Mycological Society __________________________________________________________________________________________________________ Stereum hirsutum Hairy Parchment Stereum ostrea False Turkey-tail Stereum striatum Silky Parchment Strobilurus esculentus Spruce Cone Cap Trametes gibbosa Lenzites elegans/Trametes elegans/Lenzites gibbosa Trametes hirsuta Hairy Turkey Tail Trametes versicolor Turkey-tail Trichaptum biforme Violet Toothed Polypore Trichia favoginea Physcia stellaris Star Rosette Lichen They’re coming . and hopefully they’ll bring friends! (tentative) https://siskiyou.sou.edu/2015/04/08/morel-mushrooms-the-new-gold- rush/ ESF Masters student Brandon Haynes shared the results of his research using oyster mushroom spawn to filter E April Recap coli from waste water. Many thanks to Brandon for getting the year off to a great start with his interesting Thanks to Paula Desanto for providing the following program! species list from the winter foray at the Rand Tract in March: Next month Bernie Carr will educate us about trees and Daedaleopsis confragosa Thin-maze Flat Polypore the mushrooms they grow with. A must for all Fomes fomentarius Tinder Polypore mushroom hunters! The May foray will be at Morgan Irpex lacteus Milk-white Toothed Polypore Hill State Forest . Directions : from I-81S take the Tully Ischnoderma resinosum Resinous Polypore Exit and turn left from the exit ramp. Take the next left Panellus stipticus Luminescent Panellus Schizophyllum commune Common Split Gill onto Route 80. Follow Route 80 east through Tully and Stereum complicatum Crowded Parchment Apulia. Just beyond Venture Farms take a right onto Stereum hirsutum Hairy Stereum Herlihy Road. Follow this to the top of the hill and Stereum striatum Silky Parchment turn left (before Spruce Pond). -
Aerobic Cometabolism of Chlorinated Aliphatic Hydrocarbons by Subsurface Microbes Grown on Methane, Propane and Butane from the Mcclellan Air Force Base
AN ABSTRACT OF THE THESIS OF Adisorn Tovanabootr for the degree of Masters of Science in Civil Engineering presented on April 23, 1997. Title: Aerobic Cometabolism of Chlorinated Aliphatic Hydrocarbons by Subsurface Microbes Grown on Methane, Propane and Butane from the McClellan Air Force Base. Abstract approved: Lewis Semprini Subsurface microorganisms from the McClellan Air Force Base were grown in batch aquifer microcosms on methane, propane, and butane as gaseous cometabolic substrates. The potential for aerobic cometabolism of chlorinated aliphatic hydrocarbons including trichloroethylene (TCE), 1,1,1-trichloroethane (1,1,1-TCA), and chloroform (CF) was determined. Stimulation of microorganisms on all of the substrates tested indicates a diverse microbial community exists in the McClellan subsurface. Indigenous methane and propane-utilizers were capable of transforming TCE and CF. Propane- utilizers very effectively transformed TCA, while methane-utilizers could not transform 1,1,1-TCA. The butane-utilizers were not able to degrade any of the CAHs tested. TCE was transformed most rapidly during the period of active methane consumption, and continued at a slower rate for about 1 weeks after methane was consumed. The propane culture remained active for up to four weeks after propane was consumed, and the rate followed first order kinetics. Different TCE transformation yields (Tv) (mg CAH/mg substrate) developed in replicate microcosms with time. Changes in TCE transformation ability resulted from changes in TCE concentration or TCE product toxicity. Both methane and propane-utilizers showed a positive correlation between initial TCE transformation rates and primary substrate utilization rates. The ratio of the zero order TCE transformation rate to primary substrate utilization rate was directly proportional to the ultimate transformation yield. -
Biochemistry and Physiology of Halorespiration by Desulfitobacterium Dehalogenans
Biochemistry and Physiology of Halorespiration by Desulfitobacterium dehalogenans ..?.^TJ?*LE_ LANDBOUWCATALOGU S 0000 0807 1728 Promotor: Dr.W.M . deVo s hoogleraar in de microbiologie Co-promotoren: Dr.ir .A.J.M .Stam s universitair hoofddocent bij deleerstoelgroe p Microbiologie Dr.ir . G. Schraa universitair docent bij deleerstoelgroe p Microbiologie Stellingen 1. Halorespiratie is een weinig efficiente wijze van ademhalen. Dit proefschrift 2. Halorespiratie moet worden opgevat als verbreding en niet als specialisatie van het genus Desulfitobacterium. Dit proefschrift 3. Reductieve dehalogenases zijn geen nieuwe enzymen. 4. 16S-rRNA probes zijn minder geschikt voor het aantonen van specifieke metabole activiteiten in een complex ecosysteem. Loffler etal. (2000) AEM66 : 1369;Gottscha l &Kroonema n (2000)Bode m3 : 102 5. Het "twin-arginine" transportsysteem wordt niet goed genoeg begrepen om op basis van het voorkomen van het "twin-arginine" motief enzymen te lokaliseren. Berks etal. (2000)Mol .Microbiol .35 : 260 6. Asbesthoudende bodem is niet verontreinigd. 7. Biologische groente is een pleonasme. Stellingen behorende bij het proefschrift 'Biochemistry and physiology of halorespiration by Desulfitobacterium dehalogenans' van Bram A. van de Pas Wageningen, 6 december 2000 MJOQ^O \lZ°]0 ^ Biochemistry and Physiology of Halorespiration by Desulfitobacterium dehalogenans BramA. van de Pas Proefschrift ter verkrijging van de graad van doctor op gezag van derecto r magnificus van Wageningen Universiteit, dr. ir. L. Speelman, in het openbaar -
Microbial Degradation of Chlorinated Dioxins
Available online at www.sciencedirect.com Chemosphere 71 (2008) 1005–1018 www.elsevier.com/locate/chemosphere Review Microbial degradation of chlorinated dioxins Jim A. Field *, Reyes Sierra-Alvarez Department of Chemical and Environmental Engineering, University of Arizona, P.O. Box 210011, Tucson, AZ 85721, USA Received 18 June 2007; received in revised form 30 September 2007; accepted 18 October 2007 Available online 20 February 2008 Abstract Polychlorinated dibenzo-p-dioxins (PCDD) and polychlorinated dibenzofurans (PCDF) were introduced into the biosphere on a large scale as by-products from the manufacture of chlorinated phenols and the incineration of wastes. Due to their high toxicity they have been the subject of great public and scientific scrutiny. The evidence in the literature suggests that PCDD/F compounds are subject to biodegradation in the environment as part of the natural chlorine cycle. Lower chlorinated dioxins can be degraded by aerobic bacteria from the genera of Sphingomonas, Pseudomonas and Burkholderia. Most studies have evaluated the cometabolism of monochlorinated dioxins with unsubstituted dioxin as the primary substrate. The degradation is usually initiated by unique angular dioxygenases that attack the ring adjacent to the ether oxygen. Chlorinated dioxins can also be attacked cometabolically under aerobic conditions by white-rot fungi that utilize extracellular lignin degrading peroxidases. Recently, bacteria that can grow on monochlorinated dibenzo- p-dioxins as a sole source of carbon and energy have also been characterized (Pseudomonas veronii). Higher chlorinated dioxins are known to be reductively dechlorinated in anaerobic sediments. Similar to PCB and chlorinated benzenes, halorespiring bacteria from the genus Dehalococcoides are implicated in the dechlorination reactions. -
Stebbins Cold Canyon Mushroom List List of Mushrooms Found by Bob and Barbara Sommer at the Stebbins Reserve 1985-2002
Stebbins Cold Canyon Mushroom List List of mushrooms found by Bob and Barbara Sommer at the Stebbins Reserve 1985-2002. Time sampling was not systematic and there were major gaps between visits. Some of the species names have changed since the list was started; e.g. many Hygrocybes are now Hypholoma. Number found of a species is indicated by asterisks: *** abundant (> 30 specimens) ** many specimens (10-29) * few specimens (>10) In a few cases, records of time found and number found are missing. Nov/Dec January February March/April May/June Agaricus campestris * * * Agaricus hondensis * Agaricus semotus * Agaricus silvicola * Agaricus xanthdermus * Agrocybe pediades * Agrocybe praecox * Aleuria aurantiaca * Aleuria sp. Amanita calyptrata ** * * Amanita gemmata * Amanita inversa * Amanita ocreata * * Amanita pachycolea * * * Amanita phalloides * Amanita velosa *** Armillaria mellea * Armillaria ponderosa * Anthracobia melaloma *** Armillaria ponderosa * Astraeus hygrometricus * Bolbitius vitellinus * * * * Boletus amygdalinus * Boletus appendiculatus * Boletus flaviporus * Boletus rubripes * Boletus satanus * Boletus subtomentosus * Bovista plumbea ** * Clavaria vermicularis Clavariadelphus pistillaris * Clitocybe brunneocephala * Clitocybe dealbata * Clitocybe deceptiva ** ** * * Clitocybe inversa * Clitocybe sauveolens * Collybia dryophilia * * Collybia fuscopurpurea * Collybia sp * * Coprinus disseminatus * Coprinus domesticus * Coprinus impatiens * Coprinus micaceus * * Coprinus plicatilis * * Cortinarius collinitus * Cortinarius multiformis -
Technical Protocol for Evaluating Natural Attenuation of Chlorinated
United States Office of Research and EPA/600/R-98/128 Environmental Protection Development September 1998 Agency Washington DC 20460 Technical Protocol for Evaluating Natural Attenuation of Chlorinated Solvents in Ground Water TECHNICAL PROTOCOL FOR EVALUATING NATURAL ATTENUATION OF CHLORINATED SOLVENTS IN GROUND WATER by Todd H. Wiedemeier Parsons Engineering Science, Inc. Pasadena, California Matthew A. Swanson, David E. Moutoux, and E. Kinzie Gordon Parsons Engineering Science, Inc. Denver, Colorado John T. Wilson, Barbara H. Wilson, and Donald H. Kampbell United States Environmental Protection Agency National Risk Management Research Laboratory Subsurface Protection and Remediation Division Ada, Oklahoma Patrick E. Haas, Ross N. Miller and Jerry E. Hansen Air Force Center for Environmental Excellence Technology Transfer Division Brooks Air Force Base, Texas Francis H. Chapelle United States Geological Survey Columbia, South Carolina IAG #RW57936164 Project Officer John T. Wilson National Risk Management Research Laboratory Subsurface Protection and Remediation Division Ada, Oklahoma NATIONAL RISK MANAGEMENT RESEARCH LABORATORY OFFICE OF RESEARCH AND DEVELOPMENT U. S. ENVIRONMENTAL PROTECTION AGENCY CINCINNATI, OHIO 45268 i NOTICE The information in this document was developed through a collaboration between the U.S. EPA (Subsurface Protection and Remediation Division, National Risk Management Research Laboratory, Robert S. Kerr Environmental Research Center, Ada, Oklahoma [SPRD]) and the U.S. Air Force (U.S. Air Force Center for Environmental Excellence, Brooks Air Force Base, Texas [AFCEE]). EPA staff were primarily responsible for development of the conceptual framework for the approach presented in this document; staff of the U.S. Air Force and their contractors also provided substantive input. The U.S. Air Force was primarily responsible for field testing the approach presented in this document. -
Cometabolic Degradation of Polycyclic Aromatic Hydrocarbons (Pahs) and Aromatic Ethers by Phenol- and Ammonia-Oxidizing Bacteria
AN ABSTRACT OF THE DISSERTATION OF Soon Woong Chang for the degree of Doctor of Philosophy in Civil Engineering presented on November 11, 1997. Title: Cometabolic Degradation of Polycyclic Aromatic Hydrocarbons (PAHs) and Aromatic Ethers by Phenol- and Ammonia-Oxidizing Bacteria Redacted for Privacy Abstract approved: /Kenneth J. Williamson Cometabolic biodegradation processes are potentially useful for the bioremediation of hazardous waste sites. In this study the potential application of phenol- oxidizing and nitrifying bacteria as "priming biocatalysts" was examined in the degradation of polycyclic aromatic hydrocarbons (PAHs), aryl ethers, and aromatic ethers. We observed that a phenol-oxidizing Pseudomonas strain cometabolically degrades a range of 2- and 3-ringed PAHs. A sequencing batch reactor (SBR) was used to overcome the competitive effects between two substrates and the SBR was evaluated as a alternative technology to treat mixed contaminants including phenol and PAHs. We also have demonstrated that the nitrifying bacterium Nitrosomonas europaea can cometabolically degrade a wide range polycyclic aromatic hydrocarbons (PAHs), aryl ethers and aromatic ethers including naphthalene, acenaphthene, diphenyl ether, dibenzofuran, dibenzo-p-dioxin, and anisole. Our results indicated that all the compounds are transformed by N. europaea and that several unusual reactions are involved in these reactions. In the case of naphthalene oxidation, N. europaea generated predominantly 2 naphthol whereas other monooxygenases generate 1-naphthol as the major product. In the case of dibenzofuran oxidation, 3-hydroxydibenzofuran initially accumulated in the reaction medium and was then further transformed to 3-hydroxy nitrodibenzofuran in a pH- and nitrite-dependent abiotic reaction. A similar abiotic transformation reaction also was observed with other hydroxylated aryl ethers and PAHs. -
A Model of in Situ Bioremediation That Includes the Effect of Rate- Limited Sorption and Bioavailability J
A MODEL OF IN SITU BIOREMEDIATION THAT INCLUDES THE EFFECT OF RATE- LIMITED SORPTION AND BIOAVAILABILITY J. Huang and M.N. Goltz Department of Engineering and Environmental Management, Air Force Institute of Technol- ogy, Wright-Patterson Air Force Base, OH 45433-7765; Phone: (937) 255-2998, Fax: (937) 656-4699 ABSTRACT In situ bioremediation is a groundwater and soil remediation technology that makes use of indigenous or introduced microorganisms to degrade contaminants in the subsurface. One factor affecting the feasibility of in situ bioremediation is the availability of the contaminant to the microorganisms. It is typically assumed that the contaminant is only available for biodegradation in the aqueous phase. However, many organic contami- nants of interest are sorbed to soil in the subsurface, and the sorbed contaminant is presumably unavailable for bioremediation. If bioremediation is attempted, the rate of desorption of contaminant from the sorbed phase is a factor in determining the availability of the contaminant to the degrading microorganisms and hence, the efficacy of the remediation. In this work, a transport model is presented that accounts for both biodegradation and desorption kinetics. The model is run using realistic parameter values obtained from a recent field evaluation of in situ aerobic cometabolic bioremediation at Edwards AFB, to demonstrate the potential impact of rate-limited sorption on bioremediation efficacy. The model allows remediation managers to better understand the impact of the in situ bioremediation technology on the fate and transport of contaminants, ultimately helping these managers design and implement solutions to contamination problems. Key words: modeling, bioavailability, sorption, cometabolism, bioremediation BACKGROUND In situ bioremediation is a technology that is currently being studied (and increasingly imple- mented) in the hope that it may be useful in remediating the nations hazardous waste sites. -
Electron Donor and Acceptor Utilization by Halorespiring Bacteria'
Electron donor andaccepto r utilizationb y halorespiring bacteria Maurice L.G.C. Luijten CENTRALS LANDBOUWCATALOGUS 0000 0950 9080 Promotoren Prof.dr .W.M . de Vos Hoogleraar ind eMicrobiologi c Wageningen Universiteit Prof.dr .ir .A.J.M .Stam s Persoonlijk hoogleraar bij hetlaboratoriu mvoo r Microbiologic Laboratorium voor Microbiologic, Wageningen Universiteit Copromotoren Dr.G .Schra a Universitair docent Laboratorium voor Microbiologic, Wageningen Universiteit Dr.A.A.M . Langenhoff Senior researcher/project leader Milieubiotechnologie, TNO-MEP Promotiecommissie Prof. E.J.Bouwe r John's Hopkins University, Baltimore, USA Prof.dr .C .Hollige r EPFL, Lausanne, Switzerland Dr.F .Volkerin g Tauw bv, Deventer, Nederland Prof. dr.ir .W.H . Rulkens Wageningen Universteit Dit onderzoek is uitgevoerd binnen de onderzoekschool SENSE (Netherlands Research School forth e Socio-Economic andNatura l Sciences ofth e Environment). LV''-:- v. •:-:::'.Ifir Electron donor and acceptor utilizationb y halorespiring bacteria Maurice L.G.C.Luijte n Proefschrift Terverkrijgin g van de graadva n doctor opgeza gva n derecto r magnificus van Wageningen Universiteit, prof. dr. ir. L. Speelman, inhe t openbaar te verdedigen opvrijda g 11 juni 2004 desnamiddag s te half twee in deAula . \ '\ i ocXi ^ Electron donor and acceptor utilization byhalorespirin g bacteria Maurice L.G.C. Luijten Ph.D.thesi sWageninge n University, Wageningen, The Netherlands 2004 ISBN 90-5804-067-1 Front cover: Modified EMpictur e ofSulfurospirillum halorespirans PCE-M2 — ry ' Stellingen 1.El k nadeel heb zijn voordeel. Dit proefschrift. 2. De ene volledige reductie van PCE is de andere nog niet. Dit proefschrift. 3. Sectorale communicatie reikt niet ver genoeg. HRH the Prince of Orange, Wat. -
Detection of Dehalococcoides Spp. by Peptide Nucleic Acid Fluorescent in Situ Hybridization
This article was published in Journal of Molecular Microbiology and Biotechnology, 24, 142- 149, 2014 http://dx.doi.org/10.1159/000362790 Detection of Dehalococcoides spp. by Peptide Nucleic Acid Fluorescent in situ Hybridization Anthony S. Dankoa, c Silvia J. Fonteneteb Daniel de Aquino Leiteb, e Patrícia O. Leitãoa,c Carina Almeidab, d Charles E. Schaeferf Simon Vainbergf Robert J. Steffanf Nuno F. Azevedob aCentro de Investigação em Geo-Ambiente e Recursos (CIGAR), Departamento de Engenharia de Minas, Faculdade de Engenharia, and bLaboratory for Process Engineering, Environment, and Energy and Biotechnology Engineering (LEPABE), Department of Chemical Engineering, Faculty of Engineering, University of Porto, Porto, cCentro de Recursos Naturais e Ambiente (CERENA), Instituto Superior Técnico, Lisboa, and dInstitute for Biotechnology and Bioengineering (IBB), Center of Biological Engineering, Universidade do Minho, Braga, Portugal; eLANASE, Departamento de Engenharia de Alimentos, Faculdade de Engenharia, Universidade Federal da Grande Dourados, Dourados, Brazil; fCB&I Federal Services, LLC., Lawrenceville, N.J., USA Abstract Chlorinated solvents including tetrachloroethene (perchloroethene and trichloroethene), are widely used industrial solvents. Improper use and disposal of these chemicals has led to a widespread contamination. Anaerobic treatment technologies that utilize Dehalococcoides spp. can be an effective tool to remediate these contaminated sites. There- fore, the aim of this study was to develop, optimize and validate peptide nucleic acid (PNA) probes for the detection of Dehalococcoides spp. in both pure and mixed cultures. PNA probes were designed by adapting previously published DNA probes targeting the region of the point mutations de- scribed for discriminating between the Dehalococcoides spp. strain CBDB1 and strain 195 lineages.