A New Simpler Way to Obtain High Fusion Power Gain in Tandem Mirrors
Total Page:16
File Type:pdf, Size:1020Kb
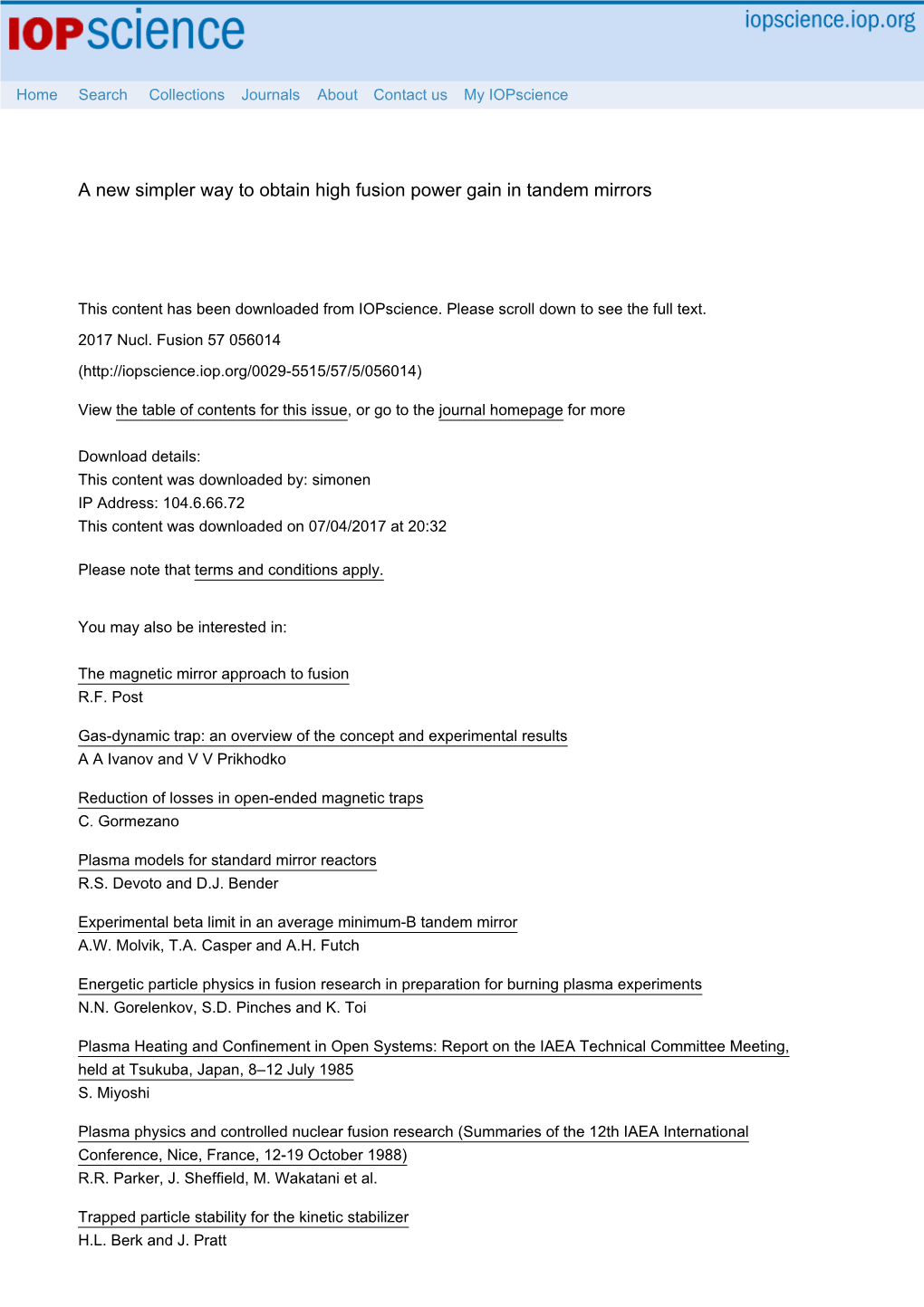
Load more
Recommended publications
-
Nuclear Weapons Databook
Nuclear Weapons Databook Volume I11 U.S. Nuclear Warhead Facility Profiles Nuclear Weapons Databook Volume I11 U.S. Nuclear Warhead Facility Profiles Thomas B. Cochran, William M. Arkin, Robert S. Morris, and Milton M. Hoenig A book by the Natural Resources Defense Council, Inc. BALUNGER PUBLISHING COMPANY Cambridge, Massachusetts A Subsidiary of Harper & Row, Publishers, Inc. Copyright a 1987 by the Natural Resources Defense Council, Inc. All rights reserved. No part of this publication may be reproduced, stored in a retrieval system, or trans- mitted in any form or by any means, electronic, mechanical, photocopy, recording or otherwise, without the prior written consent of the publisher. International Standard Book Number: 0-88730-126-6 (CL) 0-88730-146-0 (PB) Library of Congress Catalog Card Number: 82-24376 Printed in the United States of America Library of Congress CataloGng-iii-PublicationData U.S. nuclear warhead facility profiles. (Nuclear weapons databook ;v. 3) "A book by the Natural Resources Defense Council, Inc." Includes bibliographical references and index. 1. Nuclear weapons-United States. 2. Munitions-United States. I. Cochran, Thomas B. 11. Natural Resources Defense Council. 111. Title: US nuclear warhead facility profiles. IV. Title: United States nuclear warhead facility profiles. V. Series: Cochran, Thomas B. Nuclear weapons databook ;v. 3. U264.C6 1984 vol. 3 355.8'25119'0973 87-14552 [U264] ISBN 0-88410-172-X (v. 1) ISBN 0-88410-173-8 (pbk. : v. 1) ISBN 0-88730-124-X (v. 2) ISBN 0-88730-125-8 (pbk. : v. 2) ISBN 0-88730-126-6 (v. 3) ISBN 0-88730-146-0 (pbk. -
A 14 Mev Neutron Source Based on Gas Dynamic Trap Concept. Status and Perspectives E.P
30th EPS Conference on Contr. Fusion and Plasma Phys., St. Petersburg, 7-11 July 2003 ECA Vol. 27A, P-2.189 A 14 MeV Neutron Source Based on Gas Dynamic Trap Concept. Status and Perspectives E.P. Kruglyakov, A.A. Ivanov, Yu.A. Tsidulko Budker Institute of Nuclear Physics, 630090, Novosibirsk, Russia Besides the main physical problems of controlled fusion such as physics of alpha particles, ignition, etc, there exists a problem of a limited lifetime of structural materials under intensive bombardment by 14 MeV neutrons, degradations of their electrical and mechanical properties, accumulation of impurities, etc. At present, the development of the world fusion program is concentrated on the ITER project. However, even in the case of success of this project it cannot serve a function of high power neutron source for candidate material tests for future fusion power plant because of low fluence. Indeed, irradiation of one of the existing candidates on the role of a material for the first wall (ferritic-martensitic steel) with 14 MeV neutron flux of order of 2 MW/m2 during 10 years will lead to the level of radiation damages of 200 dpa. The ITER will provide only about 2 dpa per year. Thus, the fusion reactor cannot be built without performing extensive program dedicated to qualify the materials to be used in the reactor core under high level irradiation by 14 MeV and secondary neutrons. In other words, the final goal of the controlled fusion program can not be achieved without urgent design and construction of a dedicated high power 14 MeV neutron source (NS). -
Magnetic Confinement Fusion
Magnetic confinement fusion - Wikipedia 1 of 7 Magnetic confinement fusion Magnetic confinement fusion is an approach to generate thermonuclear fusion power that uses magnetic fields to confine fusion fuel in the form of a plasma. Magnetic confinement is one of two major branches of fusion energy research, along with inertial confinement fusion. The magnetic approach began in the 1940s and absorbed the majority of subsequent development. Fusion reactions combine light atomic nuclei such as hydrogen to form heavier ones such as helium, producing energy. In order to overcome the electrostatic repulsion between the nuclei, they must have a temperature of tens of millions of degrees, The reaction chamber of the TCV, an creating a plasma. In addition, the plasma experimental tokamak fusion reactor at École must be contained at a sufficient density for polytechnique fédérale de Lausanne, Lausanne, Switzerland which has been used in research a sufficient time, as specified by the Lawson since it was built in 1992. The characteristic torus- criterion (triple product). shaped chamber is clad with graphite to help withstand the extreme heat (the shape is distorted Magnetic confinement fusion attempts to by the camera's fisheye lens). use the electrical conductivity of the plasma to contain it through interaction with magnetic fields. The magnetic pressure offsets the plasma pressure. Developing a suitable arrangement of fields that contain the fuel without excessive turbulence or leaking is the primary challenge of this technology. Contents History Plasma Types Magnetic mirrors Toroidal machines Z-pinch Stellarators https://en.wikipedia.org/wiki/Magnetic_confinement_fusion Magnetic confinement fusion - Wikipedia 2 of 7 Tokamaks Compact toroids Other Magnetic fusion energy See also References External links History The development of magnetic fusion energy (MFE) came in three distinct phases. -
Axisymmetric Tandem Mirror D-T Neutron Source
Axisymmetric Tandem Mirror D-T Neutron Source W. Horton∗ and J. Pratt University of Texas at Austin, Institute for Fusion Studies A.W. Molvik Lawrence Berkeley National Laboratory R.M. Kulsrud, N.J. Fisch Princeton Plasma Physics Laboratory I. OVERVIEW We propose to harness an axisymmetric tandem mirror machine to create an intense neutron source[1] [2]. Our machine would be initially based on the successful GAMMA-10 tandem mirror experiment in Tsukuba, Japan and the Gas Dynamic Trap (GDT) mirror experiment in Novosibirsk, Russia. In addition it would be suitable for testing of several other axisymmetric stabilization methods: kinetic stabilizers [3] [4], sloshing ions, and wall stabilization, which would enable higher-Q operation than with GDT-type stabilization. The GAMMA-10 has achieved record breaking confinement results that extrapolate to the production of fusion power in a range of fusion Q from 0.1 to 5 in the near future [5]. In this work the authors outline a linear system that could produce large neutron fluxes in a spectrum nearly identical to that of the ITER device currently being built[6]. A hydrogen prototype of a D-T mirror neutron source would allow experiments on stabilization in axisymmetric cells and expanders that would lead to an optimized fusion Q design. Optimizations are needed for fusion-fission hybrid designs versus pure fusion designs. II. ADVANTAGES OF TANDEM MIRRORS The linear axisymmetric system has major conceptual advantages over all toroidal systems. We list a few of these advantages here: (1) Simplicity of engineering with no interlinked magnetic-field coils and no volt-second transformer considerations. -
Preparation and Submission of a Manuscript for the Proceedings
1 OV/P-07 Fusion Prospects of Axisymmetric Magnetic Mirror Systems A.D.Beklemishev1,2, A.V.Burdakov1,3, A.A.Ivanov1,2, E.P.Kruglyakov 1 1Budker Institute of Nuclear Physics of SB RAS, Novosibirsk, Russia 2Novosibirsk State University, Novosibirsk, Russia 3Novosibirsk State Technical University, Novosibirsk, Russia E-mail: [email protected] Abstract. Studies of magnetic mirrors for fusion started 60 years ago. The evolution of mirrors was driven by needs to stabilize curvature-driven instabilities and reduce axial losses. It turned them into highly sophisticated and huge tandem mirrors with axial ambipolar confinement, thermal barriers and quadrupole magnetic stabilizers [1,2]. Too late it was recognized that quadrupole fields cause resonant radial losses, and that placing stabilizers behind barriers reduces their effectiveness. Tandem mirrors lost competition to toroidal devices in 1987 and are almost extinct. A side branch of open traps went for simplicity, high-β and good fast-ion confinement inherent in axially symmetric mirrors [3]. These traits allow lower cost of construction and servicing, lower engineering and materials demands, promise a path to advanced-fuels fusion. Axially symmetric mirrors are of particular interest as neutron sources or fusion-fission hybrids. They might still have an edge as pure fusion reactors. Axially symmetric mirrors at the Budker Institute of Nuclear Physics in Novosibirsk currently represent the frontline of mirror research. We discuss recent experimental results from the multiple-mirror trap, GOL-3 [4], and the gas- dynamic trap, GDT [5]. The next step in this line of research is the GDMT program that will combine the GDT- style fast-ion-dominated central mirror with multiple-mirror end plugs [6]. -
Emerging Concepts Reactor Subgroup Summary J
LA-UR-99-5178 Emerging Concepts Reactor Subgroup Summary J. Hammer and R. Siemon Emerging Concepts offer unique reactor features, which may lead to a qualitative improvement in cost and maintainability, with associated increased attractiveness to the customer. Table 3 shows some of these unique features grouped by concept: Concept Motivation RFP Low external field; no disruptions Spheromak, FRC Simple geometry; small size; open axial divertor MTF, Flow Pinch Low development cost; compatible with liquid walls Levitated Dipole, Centrifugally confined High β, classical confinement; no current drive Mirrors Low physics risk; linear geometry Electrostatic, IEC, POPS Small unit size; low-cost development; high mass power density; alternate applications Fast Igniter High gain; low recirculating power Table 3: Reactor features of Emerging Concepts. Again, many examples of reactor advantages associated with Emerging Concepts could be given. As one such, there appears at first examination to be a greater accessibility for incorporating liquid walls into many such reactors. This follows from the linear, open geometry of several of the concepts, including FRC, MTF, Flow Pinch, and Mirrors. Organization The reactor subgroup, jointly with the Physics subgroup, heard presentations arranged before the conference on the 11 concepts listed below. These covered a wide range in physical parameter space with radically different reactor embodiments. The reversed field pinch and spheromak talks were held jointly with the Magnetic Confinement sessions. For each concept, a presenter introduced the concept and reviewed progress to date and important physics and reactor issues. The presenter was followed by a reviewer who brought additional insights. Concept Presenter Reviewer RFP S. -
STATUS of FUSION RESEARCH and IMPLICATIONS for D-3He SYSTEMS
STATUS OF FUSION RESEARCH AND IMPLICATIONS FOR D-3He SYSTEMS George H. Miley Fusion Studies Laboratory University of Illinois 103 South Goodwin Avenue Urbana, IL 61801 World-wide programs in both magnetic confinement and inertial confinement fusion research have made steady progress towards the experimental demonstration of energy breakeven.(*-') Both approaches are now in reach of this goal within the next few years using a D-T equivalent plasma. For magnetic confinement, this step is expected in one of the large tokamak experimental devices such as TFTR (USA), JET (EC), JT-60 (Japan), or T-15 (USSR). Upgraded versions of the Nova glass laser (USA) and CEKKO (Japan) also appear to have a good chance at this goal. The light-ion beam facility "PBFA-11" is viewed as a "dark horse" candidate. Recent physics parameters obtained in these various experiments will be briefly reviewed in this presentation. However, after breakeven is achieved, considerable time and effort must still be expended to develop a usable power plant. The time schedules envisioned by workers in the various countries involved are fairly similar.(l-J) For example, the European Community (EC) proposes to go from the physics studies in JET to an engineering test reactor (NET) which has a construction decision in 1991. This is projected to result in a demonstration reactor after 2015. Plans for inertial confinement are-currently centered on the development of a "next-step'' target facility based on an advanced 5-megajoule laser on roughly the same time scale as NET.(5) The facilities required for both magnetic and inertial confinement will be large and expensive. -
A Fusion Pioneer Talks About Fusion and How to Get There
INTERVIEW: RICHARD F. POST A Fusion Pioneer Talks About Fusion and How to Get There Dr. Post was interviewed by Managing Editor Marjorie Mazel magnetic bearing, and your flywheel idea—and anything else Hecht on June 12, 2009. you’d like to talk about. Well, fire away. Question: I’m honored to interview you Dr. Post. Reading over all your accomplishments, I think we might we need two inter- Question: We also work with a Youth Movement, and I want to views in order to ask you all the questions I have! have the youth get acquainted with some of these technolo- Our magazine, as you know, is the successor to Fusion mag- gies that have been your mission in your career. I’d like to start azine, and we have promoted fusion and advanced technolo- with fusion, and have you talk about your idea for the ATM, gies for many years now, so what I would like to cover in the the Axisymmetric Tandem Mirror fusion reactor. You’ve been interview is the fusion question, the Inductrack maglev, the working on this for a long time. How do we bring this into be- ing? In the first place, I would not call it my idea. I did come up with a way of doing it, but there are many ways to skin a cat. The basic concept, that is not what I came up with. I’d been looking at a way of making an ATM, based on theory by [Dmitri] Ryutov but as we learned, there are also many other ways to sta- bilize the MHD [magnetohy- drodynamic] instability mode of an Axisymmetric Tandem Mirror. -
The Fairy Tale of Nuclear Fusion L
The Fairy Tale of Nuclear Fusion L. J. Reinders The Fairy Tale of Nuclear Fusion 123 L. J. Reinders Panningen, The Netherlands ISBN 978-3-030-64343-0 ISBN 978-3-030-64344-7 (eBook) https://doi.org/10.1007/978-3-030-64344-7 © The Editor(s) (if applicable) and The Author(s), under exclusive license to Springer Nature Switzerland AG 2021 This work is subject to copyright. All rights are solely and exclusively licensed by the Publisher, whether the whole or part of the material is concerned, specifically the rights of translation, reprinting, reuse of illustrations, recitation, broadcasting, reproduction on microfilms or in any other physical way, and transmission or information storage and retrieval, electronic adaptation, computer software, or by similar or dissimilar methodology now known or hereafter developed. The use of general descriptive names, registered names, trademarks, service marks, etc. in this publication does not imply, even in the absence of a specific statement, that such names are exempt from the relevant protective laws and regulations and therefore free for general use. The publisher, the authors and the editors are safe to assume that the advice and information in this book are believed to be true and accurate at the date of publication. Neither the publisher nor the authors or the editors give a warranty, expressed or implied, with respect to the material contained herein or for any errors or omissions that may have been made. The publisher remains neutral with regard to jurisdictional claims in published maps and institutional affiliations. This Springer imprint is published by the registered company Springer Nature Switzerland AG The registered company address is: Gewerbestrasse 11, 6330 Cham, Switzerland When you are studying any matter or considering any philosophy, ask yourself only what are the facts and what is the truth that the facts bear out. -
American Nuclear Society
AMERICAN NUCLEAR SOCIETY . 555 North Kensington Avenue Tel: 708 / 352-6611 La Grange Park, Illinois E-Mail: [email protected] 60526-5592 USA http: //www.ans.org Fax: 708 / 352-6464 AUTHOR PROOF INSTRUCTIONS Your paper is scheduled for publication in a forthcoming issue of Nuclear Technology. You are receiving your author proofs electronically. You will not receive anything in the regular mail. Please follow these procedures: 1. Download your author proof, print it out, and review it. 2. Proofread your paper very carefully. This will be your final reading before publication. This material has been copyedited and typeset from the disk/electronic file that you provided with your manuscript or from the hard copy that you provided, and it has been transmitted to you through the Internet. This process produces excellent results, but please check the following especially carefully: translation of Greek letters, accented letters, special characters, superscripts and subscripts, and mathematical symbols; typesetting of mathematics; and formatting of tables. Also, note that your manuscript has been copyedited according to ANS technical journal style; please check that technical meanings have been unaffected by the copyediting. Please limit your corrections to those that are absolutely necessary because changes at this stage of processing are both time-consuming and expensive. 3. Please note that the typesetter has used low-resolution electronically scanned versions of your figures as FPOs (for position only). These will be replaced with high-resolution versions at the printing stage. 4. Necessary corrections should be sent as follows: (a) a list of corrections sent by electronic mail and/or (b) your manuscript with your corrections marked on it sent by fax (your fax should include a list of your corrections). -
Plasma Physics and Controlled Nuclear Fusion Research 1984 Vol.3 TENTH CONFERENCE PROCEEDINGS, LONDON, 12-19 SEPTEMBER 1984 Nuclear Fusion, Supplement 1985
Plasma Physics and Controlled Nuclear Fusion Research 1984 Vol.3 TENTH CONFERENCE PROCEEDINGS, LONDON, 12-19 SEPTEMBER 1984 Nuclear Fusion, Supplement 1985 fj&\ VW& INTERNATIONAL ATOMIC ENERGY AGENCY, VIENNA, 1985 ^^ m PLASMA PHYSICS AND CONTROLLED NUCLEAR FUSION RESEARCH 1984 VOLUME 3 The following States are Members of the International Atomic Energy Agency: AFGHANISTAN HAITI PARAGUAY ALBANIA HOLY SEE PERU ALGERIA HUNGARY PHILIPPINES ARGENTINA ICELAND POLAND AUSTRALIA INDIA PORTUGAL AUSTRIA INDONESIA QATAR BANGLADESH IRAN, ISLAMIC REPUBLIC OF ROMANIA BELGIUM IRAQ SAUDI ARABIA BOLIVIA IRELAND SENEGAL BRAZIL ISRAEL SIERRA LEONE BULGARIA ITALY SINGAPORE BURMA IVORY COAST SOUTH AFRICA BYELORUSSIAN SOVIET JAMAICA SPAIN SOCIALIST REPUBLIC JAPAN SRI LANKA CAMEROON JORDAN SUDAN CANADA KENYA SWEDEN CHILE KOREA, REPUBLIC OF SWITZERLAND CHINA KUWAIT SYRIAN ARAB REPUBLIC COLOMBIA LEBANON THAILAND COSTA RICA LIBERIA TUNISIA CUBA LIBYAN ARAB JAMAHIRIYA TURKEY CYPRUS LIECHTENSTEIN UGANDA CZECHOSLOVAKIA LUXEMBOURG UKRAINIAN SOVIET SOCIALIST DEMOCRATIC KAMPUCHEA MADAGASCAR REPUBLIC DEMOCRATIC PEOPLE'S MALAYSIA UNION OF SOVIET SOCIALIST REPUBLIC OF KOREA MALI REPUBLICS DENMARK MAURITIUS UNITED ARAB EMIRATES DOMINICAN REPUBLIC MEXICO UNITED KINGDOM OF GREAT ECUADOR MONACO BRITAIN AND NORTHERN EGYPT MONGOLIA IRELAND EL SALVADOR MOROCCO UNITED REPUBLIC OF ETHIOPIA NAMIBIA TANZANIA FINLAND NETHERLANDS UNITED STATES OF AMERICA FRANCE NEW ZEALAND URUGUAY GABON NICARAGUA VENEZUELA GERMAN DEMOCRATIC REPUBLIC NIGER VIET NAM GERMANY, FEDERAL REPUBLIC OF NIGERIA YUGOSLAVIA GHANA NORWAY ZAIRE GREECE PAKISTAN ZAMBIA GUATEMALA PANAMA The Agency's Statute was approved on 23 October 1956 by the Conference on the Statute of the IAEA held at United Nations Headquarters, New York; it entered into force on 29 July 1957. The Headquarters of the Agency are situated in Vienna. -
Fusion Devices
PP.EPRINI UCRL-S0060 r~ _ _ZZ~ j Lawrence Uvermore Laboratory FUSION DEVICES T. K. FOWLER OCTOBER 11, 197"/ Presented at the EPRI Executive Seminar on Fusion, October 11-13, 1977 San Francisco, California This is a preprint of a paper intended for publication in a Journal or proceedings. Since changes may be made before publication, this preprint is made available with the understanding that It will not be cited or reproduced without the permission of the author. )0B ©1STR1BUT10N OF THIS DOCUMENT IS UNLIMITED 1. FUSION DEVICES T. K. Fowler In this talk, I will emphasize the expected developments in fusion research over the next five years. This will be the formative period for fusion in the foreseeable future. It will also be a formative period in national energy policy that will impact many years to come. As the previous speakers have said, we believe that fusion is a matter of great interest to the electric utilities. We need your support and your guidance in this critically formative period. I will give a brief description of the three mainline activities of the research program in fusion. These include two magnetic systems, one called the Tokamak and one called the mirror machine, and also laser fusion. While I am going to concentrate on these three mainline activities, they are not the only possibilities. Fusion is a rich subject with many possible outcomes, all of which may be interesting to the utilities. However, the first ways that fusion will be made practical will, I think, come from the approaches I will describe, simply because each of these concepts already has behind it a long history of scientific development that should culminate in the next five years.