METHODS to IMPROVE the REMEDIATION of POLYCYCLIC AROMATIC HYDROCARBONS (Pahs) in AEROBIC and ANAEROBIC ENVIRONMENTS
Total Page:16
File Type:pdf, Size:1020Kb
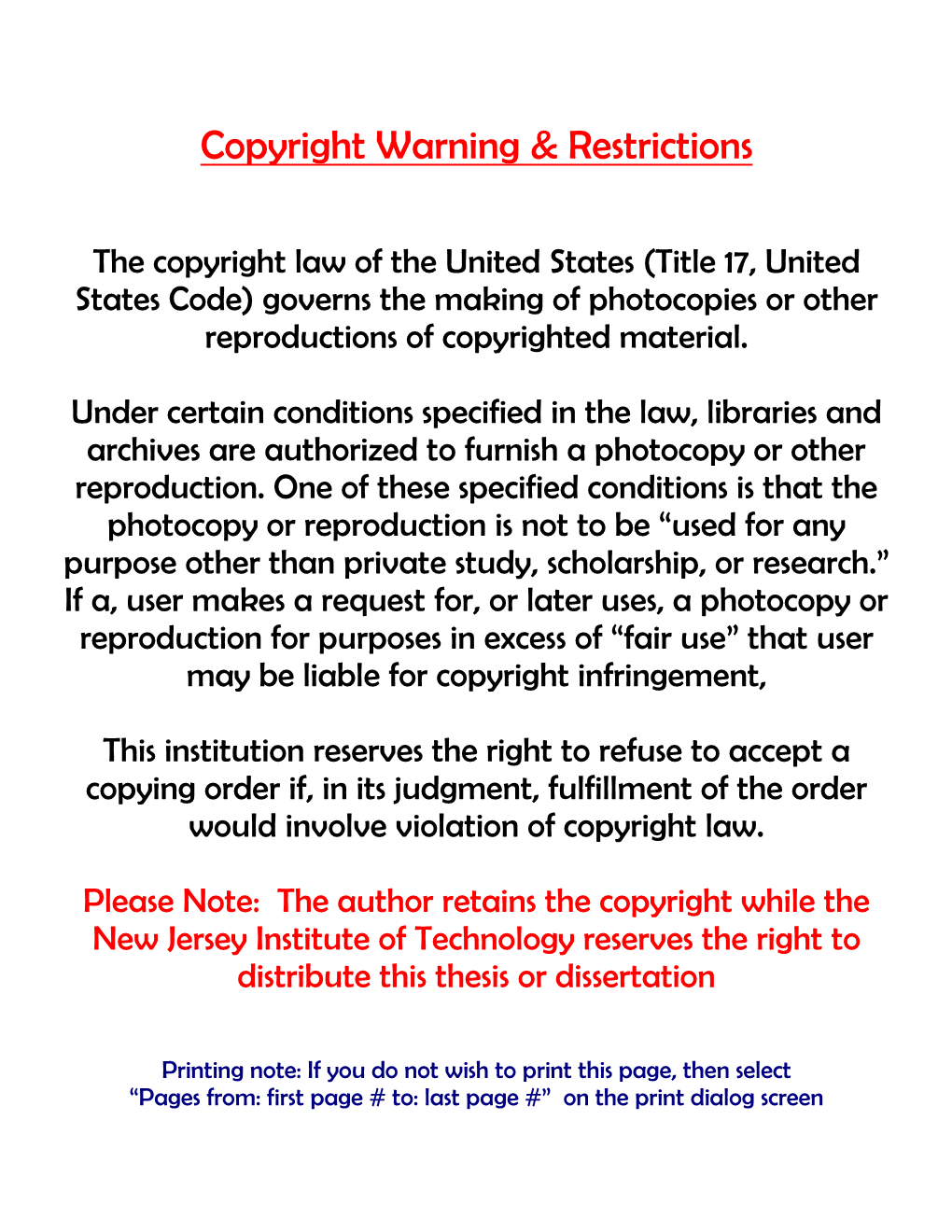
Load more
Recommended publications
-
Inferring Microbial Interaction Networks Based on Consensus Similarity Network Fusion
SCIENCE CHINA Life Sciences THEMATIC ISSUE: Computational life sciences November 2014 Vol.57 No.11: 1115–1120 • RESEARCH PAPER • doi: 10.1007/s11427-014-4735-x Inferring microbial interaction networks based on consensus similarity network fusion JIANG XingPeng1,2 & HU XiaoHua2* 1College of Computing and Informatics, Drexel University, Philadelphia, PA 19104, USA; 2School of Computer, Central China Normal University, Wuhan 430079, China Received May 15, 2014; accepted July 21, 2014; published online October 17, 2014 With the rapid accumulation of high-throughput metagenomic sequencing data, it is possible to infer microbial species rela- tions in a microbial community systematically. In recent years, some approaches have been proposed for identifying microbial interaction network. These methods often focus on one dataset without considering the advantage of data integration. In this study, we propose to use a similarity network fusion (SNF) method to infer microbial relations. The SNF efficiently integrates the similarities of species derived from different datasets by a cross-network diffusion process. We also introduce consensus k-nearest neighborhood (Ck-NN) method instead of k-NN in the original SNF (we call the approach CSNF). The final network represents the augmented species relationships with aggregated evidence from various datasets, taking advantage of comple- mentarity in the data. We apply the method on genus profiles derived from three microbiome datasets and we find that CSNF can discover the modular structure of microbial interaction network which cannot be identified by analyzing a single dataset. species interaction, metagenome, diffusion process, biological network, modularity Citation: Jiang XP, Hu XH. Inferring microbial interaction networks based on consensus similarity network fusion. -
Bioaugmentation of Chlorinated Solvents
BIOAUGMENTATION FOR REMEDIATION OF CHLORINATED SOLVENTS: TECHNOLOGY DEVELOPMENT, STATUS, AND RESEARCH NEEDS October 2005 GeoSyntec Consultants TABLE OF CONTENTS LIST OF TABLES ...........................................................................................................................................III LIST OF FIGURES .........................................................................................................................................III ACRONYMNS AND ABBREVIATIONS........................................................................................................V FOREWORD...................................................................................................................................................VII EXECUTIVE SUMMARY ............................................................................................................................... IX 1. INTRODUCTION ..........................................................................................................................................1 2. EARLY DEVELOPMENT OF BIOAUGMENTATION.............................................................................5 3. RECENT PROGRESS IN CHLORINATED SOLVENT BIOREMEDIATION .....................................13 4. DEHALORESPIRATION: THE KEY PROCESS UNDERLYING CURRENT BIOAUGMENTATION PRACTICES..............................................................................................................................................................19 4.1 THE UBIQUITY CONCEPT REVISITED -
Understanding the Interaction Between Anabaena Sp. and a Heterocyst-Specific Epibiont
Understanding the interaction between Anabaena sp. and a heterocyst-specific epibiont Iglika V. Pavlova MBL Microbial Diversity course 2005 Abstract Anabaena sp. and an associated heterocyst-specific epibiontic bacterium were isolated from School Street Marsh in Woods Hole, MA in 1997 during the Microbial Diversity course. A two-member culture of the cyanobacterium and the epibiont have been maintained at WHOI by John Waterbury. Anabaena species with similar heterocyst-specific epibionts have also been isolated from other locations (7, 8, 9). Successful culture of the epibiont separately from the School Street Marsh cyanobacterium was achieved on two occasions (1, 10), but the isolates were not preserved. The epibiont was identified to be an α-proteobacterium within the Rhizobiaceae group (10). The close and specific association between the cyanobacterium and the epibiont strongly suggests there might be a physiological benefit or benefits to the cyanobacterium, the epibiont, or to both partners. This study was designed to understand the potential benefits of the interaction for the epibiontic bacterium. Several approaches were used to isolate the epibiont in pure culture, unsuccessfully. This report describes the rationale for undertaking the project and the approaches used to isolate the epibiont, in the hopes that this will be useful information for the future isolation of an axenic epibiont culture. Rationale Cyanobacterial associations with heterotrophic bacteria from environmental isolates have been described before and have been implicated in increasing the growth of the cyanobacterium (5, 6, 7). Benefit to the cyanobacterium may be derived from a range of associations, including the presence of heterotrophic bacteria in the culture medium, attraction of such bacteria to cyanobacterial secretions (either along the whole filament, or secretions stemming from the heterocyst in filamentous bacteria), or the attachment of the bacterium to the cyanobacterium outer surface (5, 6, 7, 9). -
Escherichia Coli
log bio y: O ro p c e i n M A l c Clinical Microbiology: Open a c c i e n s i l s Delmas et al., Clin Microbiol 2015, 4:2 C Access ISSN: 2327-5073 DOI:10.4172/2327-5073.1000195 Commentary Open Access Escherichia coli: The Good, the Bad and the Ugly Julien Delmas*, Guillaume Dalmasso and Richard Bonnet Microbes, Intestine, Inflammation and Host Susceptibility, INSERM U1071, INRA USC2018, Université Clermont Auvergne, Clermont-Ferrand, France *Corresponding author: Julien Delmas, Microbes, Intestine, Inflammation and Host Susceptibility, INSERM U1071, INRA USC2018, Université Clermont Auvergne, Clermont-Ferrand, France, Tel: +334731779; E-mail; [email protected] Received date: March 11, 2015, Accepted date: April 21, 2015, Published date: Aptil 28, 2015 Copyright: © 2015 Delmas J, et al. This is an open-access article distributed under the terms of the Creative Commons Attribution License, which permits unrestricted use, distribution, and reproduction in any medium, provided the original author and source are credited. Abstract The species Escherichia coli comprises non-pathogenic commensal strains that form part of the normal flora of humans and virulent strains responsible for acute infections inside and outside the intestine. In addition to these pathotypes, various strains of E. coli are suspected of promoting the development or exacerbation of chronic diseases of the intestine such as Crohn’s disease and colorectal cancer. Description replicate within both intestinal epithelial cells and macrophages. These properties were used to define a new pathotype of E. coli designated Escherichia coli is a non-sporeforming, facultatively anaerobic adherent-invasive E. -
Frequency and Potential Dependence of Reversible Electrocatalytic Hydrogen Interconversion by [Fefe]-Hydrogenases
Frequency and potential dependence of reversible electrocatalytic hydrogen interconversion by [FeFe]-hydrogenases Kavita Pandeya,b, Shams T. A. Islama, Thomas Happec, and Fraser A. Armstronga,1 aInorganic Chemistry Laboratory, Department of Chemistry, University of Oxford, Oxford OX1 3QR, United Kingdom; bSchool of Solar Energy, Pandit Deendayal Petroleum University, Gandhinagar 382007, Gujarat, India; and cAG Photobiotechnologie Ruhr–Universität Bochum, 44801 Bochum, Germany Edited by Thomas E. Mallouk, The Pennsylvania State University, University Park, PA, and approved March 2, 2017 (received for review December 5, 2016) The kinetics of hydrogen oxidation and evolution by [FeFe]- CrHydA1 contains only the H cluster: in the living cell, it receives hydrogenases have been investigated by electrochemical imped- electrons from photosystem I via a small [2Fe–2S] ferredoxin ance spectroscopy—resolving factors that determine the excep- known as PetF (19). No accessory internal Fe–S clusters are tional activity of these enzymes, and introducing an unusual and present to mediate or store electrons. powerful way of analyzing their catalytic electron transport prop- For enzymes, PFE has focused entirely on net catalytic electron erties. Attached to an electrode, hydrogenases display reversible flow that is observed as direct current (DC) in voltammograms + electrocatalytic behavior close to the 2H /H2 potential, making (2, 3, 10). Use of DC cyclic voltammetry as opposed to single them paradigms for efficiency: the electrocatalytic “exchange” rate potential sweeps alone helps to distinguish rapid, steady-state (measured around zero driving force) is therefore an unusual pa- catalytic activity at different potentials from relatively slow, rameter with theoretical and practical significance. Experiments potential-dependent changes in activity that are revealed as were carried out on two [FeFe]-hydrogenases, CrHydA1 from the hysteresis (10). -
University of Oklahoma Graduate College Microbial Ecology of Coastal Ecosystems: Investigations of the Genetic Potential For
UNIVERSITY OF OKLAHOMA GRADUATE COLLEGE MICROBIAL ECOLOGY OF COASTAL ECOSYSTEMS: INVESTIGATIONS OF THE GENETIC POTENTIAL FOR ANAEROBIC HYDROCARBON TRANSFORMATION AND THE RESPONSE TO HYDROCARBON EXPOSURE A DISSERTATION SUBMITTED TO THE GRADUATE FACULTY in partial fulfillment of the requirements of the Degree of DOCTOR OF PHILOSOPHY By JAMIE M. JOHNSON DUFFNER Norman, Oklahoma 2017 MICROBIAL ECOLOGY OF COASTAL ECOSYSTEMS: INVESTIGATIONS OF THE GENETIC POTENTIAL FOR ANAEROBIC HYDROCARBON TRANSFORMATION AND THE RESPONSE TO HYDROCARBON EXPOSURE A DISSERTATION APPROVED FOR THE DEPARTMENT OF MICROBIOLOGY AND PLANT BIOLOGY BY ______________________________ Dr. Amy V. Callaghan, Chair ______________________________ Dr. Lee R. Krumholz ______________________________ Dr. Michael J. McInerney ______________________________ Dr. Mark A. Nanny ______________________________ Dr. Boris Wawrik © Copyright by JAMIE M. JOHNSON DUFFNER 2017 All Rights Reserved. This dissertation is dedicated to my husband, Derick, for his unwavering love and support. Acknowledgements I would like to thank my advisor, Dr. Amy Callaghan, for her guidance and support during my time as a graduate student, as well as for her commitment to my success as a scientist. I would also like to thank the members of my doctoral committee for their guidance over the years, particularly that of Dr. Boris Wawrik. I would like to also thank Drs. Josh Cooper, Chris Lyles, and Chris Marks for their friendship and support during my time at OU, both in and outside the lab. iv Table of Contents -
Computational Redox Potential Predictions: Applications to Inorganic and Organic Aqueous Complexes, and Complexes Adsorbed to Mineral Surfaces
Minerals 2014, 4, 345-387; doi:10.3390/min4020345 OPEN ACCESS minerals ISSN 2075-163X www.mdpi.com/journal/minerals Review Computational Redox Potential Predictions: Applications to Inorganic and Organic Aqueous Complexes, and Complexes Adsorbed to Mineral Surfaces Krishnamoorthy Arumugam and Udo Becker * Department of Earth and Environmental Sciences, University of Michigan, 1100 North University Avenue, 2534 C.C. Little, Ann Arbor, MI 48109-1005, USA; E-Mail: [email protected] * Author to whom correspondence should be addressed; E-Mail: [email protected]; Tel.: +1-734-615-6894; Fax: +1-734-763-4690. Received: 11 February 2014; in revised form: 3 April 2014 / Accepted: 13 April 2014 / Published: 24 April 2014 Abstract: Applications of redox processes range over a number of scientific fields. This review article summarizes the theory behind the calculation of redox potentials in solution for species such as organic compounds, inorganic complexes, actinides, battery materials, and mineral surface-bound-species. Different computational approaches to predict and determine redox potentials of electron transitions are discussed along with their respective pros and cons for the prediction of redox potentials. Subsequently, recommendations are made for certain necessary computational settings required for accurate calculation of redox potentials. This article reviews the importance of computational parameters, such as basis sets, density functional theory (DFT) functionals, and relativistic approaches and the role that physicochemical processes play on the shift of redox potentials, such as hydration or spin orbit coupling, and will aid in finding suitable combinations of approaches for different chemical and geochemical applications. Identifying cost-effective and credible computational approaches is essential to benchmark redox potential calculations against experiments. -
"Relationships Between Oxidation-Reduction Potential
Relationships between Oxidation-Reduction Potential, Oxidant, and pH in Drinking Water Cheryl N. James3, Rachel C. Copeland2, and Darren A. Lytle1 1U.S. Environmental Protection Agency, NRMRL, Cincinnati, OH 2University of Cincinnati, Department of Environmental Engineering, Cincinnati, OH 3University of Cincinnati, Department of Chemical Engineering, Cincinnati, OH Abstract –Oxidation and reduction (redox) reactions are very important in drinking water. Oxidation-reduction potential (ORP) measurements reflect the redox state of water. Redox measurements are not widely made by drinking water utilities in part because they are not well understood. The objective of this study was to determine the effect of oxidant type and concentration on the ORP of carbonate buffered water as a function of pH. Oxidants that were studied included: chlorine, monochloramine, potassium permanganate, chlorine dioxide, and oxygen. ORP decreased with increasing pH, regardless of the oxidant type or concentration. ORP increased rapidly with increasing oxidant dosage, particularly at lower concentrations. Differences in the redox potentials of different oxidant systems were also observed. Waters that contained chlorine and chlorine dioxide had the highest ORPs. Tests also revealed that there were inconsistencies with redox electrode measurements. In the standard Zobell reference solution, two identical redox electrodes had nearly the same reading, but in test waters the readings sometimes showed a variation as great as 217.7 mV. Key words: ORP, redox potential, redox chemistry, oxidant, drinking water 1.0 BACKGROUND 1.1 Redox Theory Oxidation-reduction (redox) reactions describe the transfer of electrons between atoms, molecules, or ions. Oxidation and reduction reactions occur simultaneously and together make up an electrochemical couple. -
1 Characterization of Sulfur Metabolizing Microbes in a Cold Saline Microbial Mat of the Canadian High Arctic Raven Comery Mast
Characterization of sulfur metabolizing microbes in a cold saline microbial mat of the Canadian High Arctic Raven Comery Master of Science Department of Natural Resource Sciences Unit: Microbiology McGill University, Montreal July 2015 A thesis submitted to McGill University in partial fulfillment of the requirements of the degree of Master in Science © Raven Comery 2015 1 Abstract/Résumé The Gypsum Hill (GH) spring system is located on Axel Heiberg Island of the High Arctic, perennially discharging cold hypersaline water rich in sulfur compounds. Microbial mats are found adjacent to channels of the GH springs. This thesis is the first detailed analysis of the Gypsum Hill spring microbial mats and their microbial diversity. Physicochemical analyses of the water saturating the GH spring microbial mat show that in summer it is cold (9°C), hypersaline (5.6%), and contains sulfide (0-10 ppm) and thiosulfate (>50 ppm). Pyrosequencing analyses were carried out on both 16S rRNA transcripts (i.e. cDNA) and genes (i.e. DNA) to investigate the mat’s community composition, diversity, and putatively active members. In order to investigate the sulfate reducing community in detail, the sulfite reductase gene and its transcript were also sequenced. Finally, enrichment cultures for sulfate/sulfur reducing bacteria were set up and monitored for sulfide production at cold temperatures. Overall, sulfur metabolism was found to be an important component of the GH microbial mat system, particularly the active fraction, as 49% of DNA and 77% of cDNA from bacterial 16S rRNA gene libraries were classified as taxa capable of the reduction or oxidation of sulfur compounds. -
Biogeochemical Processes and Microbial Diversity of the Gullfaks and Tommeliten Methane Seeps (Northern North Sea)
Biogeosciences, 5, 1127–1144, 2008 www.biogeosciences.net/5/1127/2008/ Biogeosciences © Author(s) 2008. This work is distributed under the Creative Commons Attribution 3.0 License. Biogeochemical processes and microbial diversity of the Gullfaks and Tommeliten methane seeps (Northern North Sea) G. Wegener1, M. Shovitri1, K. Knittel1, H. Niemann1,2,*, M. Hovland3, and A. Boetius1,2,4 1Max Planck Institute for Marine Microbiology, Bremen, Germany 2Alfred Wegener Institute for Polar and Marine Research, Bremerhaven, Germany 3Statoil, Stavanger, Norway 4Jacobs University Bremen, Bremen, Germany *now at: Institute for Environmental Geosciences, University of Basel, Switzerland Received: 21 January 2008 – Published in Biogeosciences Discuss.: 25 February 2008 Revised: 3 June 2008 – Accepted: 11 July 2008 – Published: 18 August 2008 Abstract. Fluid flow related seafloor structures and gas seeps microbial community incorporates methane or its metabo- were detected in the North Sea in the 1970s and 1980s by lites. The microbial community composition of both shallow acoustic sub-bottom profiling and oil rig surveys. A vari- seeps shows high similarities to the deep water seeps asso- ety of features like pockmarks, gas vents and authigenic car- ciated with gas hydrates such as Hydrate Ridge or the Eel bonate cements were found to be associated with sites of River basin. oil and gas exploration, indicating a link between these sur- face structures and the underlying, deep hydrocarbon reser- voirs. In this study we performed acoustic surveys and videographic observation at Gullfaks, Holene Trench, Tom- 1 Introduction meliten, Witch’s Hole and the giant pockmarks of the UK Block 15/25, to investigate the occurrence and distribution of The North Sea is a marginal sea of the Atlantic on the Eu- cold seep ecosystems in the Northern North Sea. -
High Diversity of Anaerobic Alkane-Degrading Microbial Communities in Marine Seep Sediments Based on (1-Methylalkyl)Succinate Synthase Genes
ORIGINAL RESEARCH published: 07 January 2016 doi: 10.3389/fmicb.2015.01511 High Diversity of Anaerobic Alkane-Degrading Microbial Communities in Marine Seep Sediments Based on (1-methylalkyl)succinate Synthase Genes Marion H. Stagars1,S.EmilRuff1,2† , Rudolf Amann1 and Katrin Knittel1* 1 Department of Molecular Ecology, Max Planck Institute for Marine Microbiology, Bremen, Germany, 2 HGF MPG Joint Research Group for Deep-Sea Ecology and Technology, Max Planck Institute for Marine Microbiology, Bremen, Germany Edited by: Alkanes comprise a substantial fraction of crude oil and are prevalent at marine seeps. Hans H. Richnow, These environments are typically anoxic and host diverse microbial communities that Helmholtz Centre for Environmental Research, Germany grow on alkanes. The most widely distributed mechanism of anaerobic alkane activation Reviewed by: is the addition of alkanes to fumarate by (1-methylalkyl)succinate synthase (Mas). Here Beth Orcutt, we studied the diversity of MasD, the catalytic subunit of the enzyme, in 12 marine Bigelow Laboratory for Ocean sediments sampled at seven seeps. We aimed to identify cosmopolitan species as well Sciences, USA Zhidan Liu, as to identify factors structuring the alkane-degrading community. Using next generation China Agricultural University, China sequencing we obtained a total of 420 MasD species-level operational taxonomic units *Correspondence: (OTU0.96) at 96% amino acid identity. Diversity analysis shows a high richness and Katrin Knittel [email protected] evenness of alkane-degrading bacteria. Sites with similar hydrocarbon composition harbored similar alkane-degrading communities based on MasD genes; the MasD †Present address: community structure is clearly driven by the hydrocarbon source available at the various S. -
Microbial Diversity of Soda Lake Habitats
Microbial Diversity of Soda Lake Habitats Von der Gemeinsamen Naturwissenschaftlichen Fakultät der Technischen Universität Carolo-Wilhelmina zu Braunschweig zur Erlangung des Grades eines Doktors der Naturwissenschaften (Dr. rer. nat.) genehmigte D i s s e r t a t i o n von Susanne Baumgarte aus Fritzlar 1. Referent: Prof. Dr. K. N. Timmis 2. Referent: Prof. Dr. E. Stackebrandt eingereicht am: 26.08.2002 mündliche Prüfung (Disputation) am: 10.01.2003 2003 Vorveröffentlichungen der Dissertation Teilergebnisse aus dieser Arbeit wurden mit Genehmigung der Gemeinsamen Naturwissenschaftlichen Fakultät, vertreten durch den Mentor der Arbeit, in folgenden Beiträgen vorab veröffentlicht: Publikationen Baumgarte, S., Moore, E. R. & Tindall, B. J. (2001). Re-examining the 16S rDNA sequence of Halomonas salina. International Journal of Systematic and Evolutionary Microbiology 51: 51-53. Tagungsbeiträge Baumgarte, S., Mau, M., Bennasar, A., Moore, E. R., Tindall, B. J. & Timmis, K. N. (1999). Archaeal diversity in soda lake habitats. (Vortrag). Jahrestagung der VAAM, Göttingen. Baumgarte, S., Tindall, B. J., Mau, M., Bennasar, A., Timmis, K. N. & Moore, E. R. (1998). Bacterial and archaeal diversity in an African soda lake. (Poster). Körber Symposium on Molecular and Microsensor Studies of Microbial Communities, Bremen. II Contents 1. Introduction............................................................................................................... 1 1.1. The soda lake environment .................................................................................