Studies in Microwave and RF Capacitively Coupled Excimer Lamp
Total Page:16
File Type:pdf, Size:1020Kb
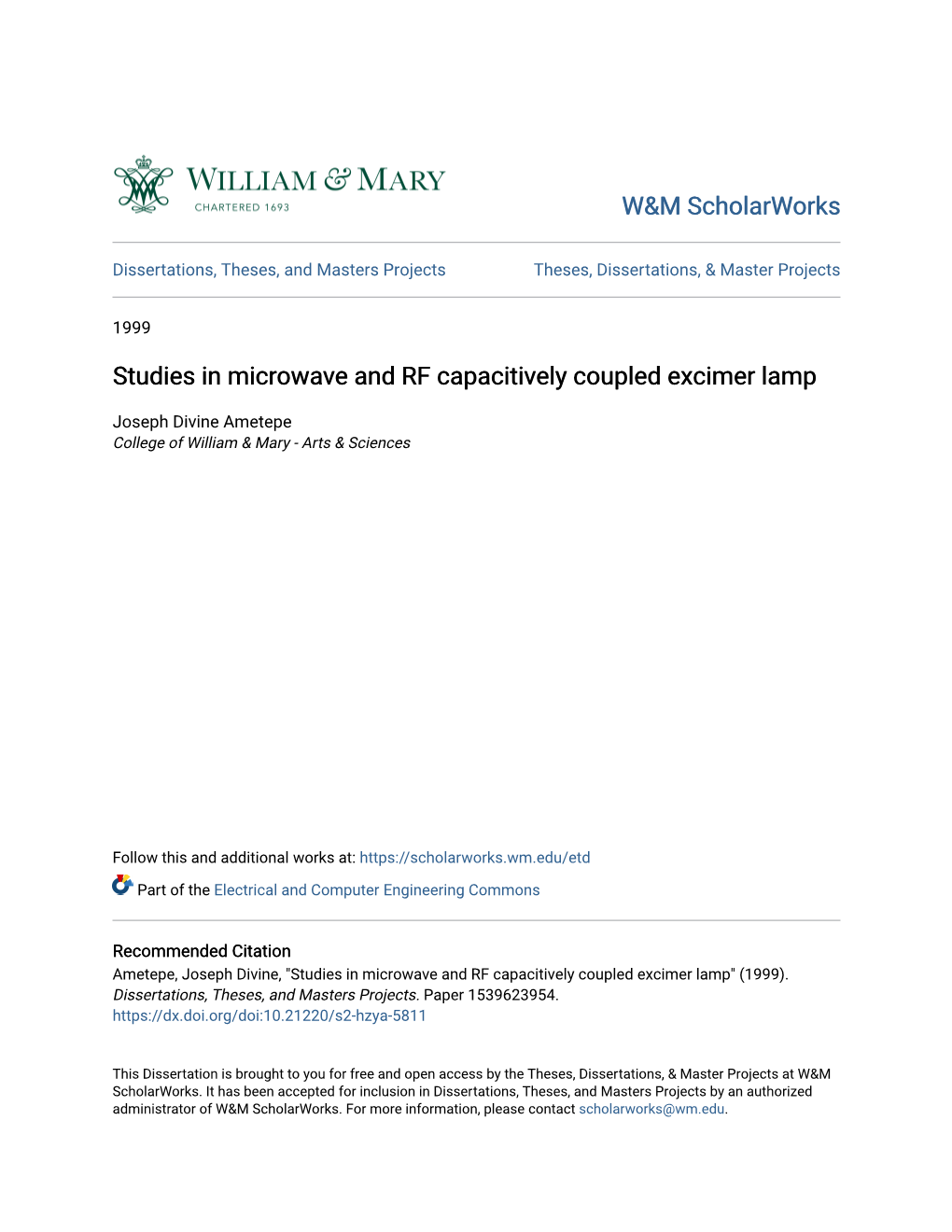
Load more
Recommended publications
-
Advanced Excimer Laser Technologies Enable Green Semiconductor Manufacturing
PROCEEDINGS OF SPIE SPIEDigitalLibrary.org/conference-proceedings-of-spie Advanced excimer laser technologies enable green semiconductor manufacturing Hitomi Fukuda, Youngsun Yoo, Yuji Minegishi, Naoto Hisanaga, Tatsuo Enami Downloaded From: https://www.spiedigitallibrary.org/conference-proceedings-of-spie on 10/25/2017 Terms of Use: https://spiedigitallibrary.spie.org/ss/TermsOfUse.aspx Advanced Excimer Laser Technologies Enable Green Semiconductor Manufacturing Hitomi Fukuda*, Youngsun Yoo, Yuji Minegishi, Naoto Hisanaga and Tatsuo Enami Gigaphoton Inc., 400 Yokokura-Shinden, Oyama-shi, Tochigi, JAPAN 323-8558 ABSTRACT "Green" has fast become an important and pervasive topic throughout many industries worldwide. Many companies, especially in the manufacturing industries, have taken steps to integrate green initiatives into their high-level corporate strategies. Governments have also been active in implementing various initiatives designed to increase corporate responsibility and accountability towards environmental issues. In the semiconductor manufacturing industry, there are growing concerns over future environmental impact as enormous fabs expand and new generation of equipments become larger and more powerful. To address these concerns, Gigaphoton has implemented various green initiatives for many years under the EcoPhoton™ program. The objective of this program is to drive innovations in technology and services that enable manufacturers to significantly reduce both the financial and environmental “green cost” of laser operations in high-volume manufacturing environment (HVM) – primarily focusing on electricity, gas and heat management costs. One example of such innovation is Gigaphoton’s Injection-Lock system, which reduces electricity and gas utilization costs of the laser by up to 50%. Furthermore, to support the industry’s transition from 300mm to the next generation 450mm wafers, technologies are being developed to create lasers that offer double the output power from 60W to 120W, but reducing electricity and gas consumption by another 50%. -
Modification Cleaning Bonding
EXCIMER LAMP LIGHT SOURCE Bonding Cleaning Modification EXCIMER LAMP LIGHT SOURCE RESULTS * Data verified by in-house testing. ■Surface modification of various materials TLS B0019EA Modification 100 Irradiation distance: 2 mm 90 Irradiation time: 10 s Irradiation atmosphere: air Surface modification technology is 80 utilized in a wide range of industrial Before processing fields. Compared to ordinary techniques, 70 After processing material modification using excimer 60 lamps is considered precision modifica- 50 tion because it occurs via a chemical reaction on the atomic or molecular level. 40 Moreover, this is clean modification that 30 does not harm the material and generates 20 no dust particles, and so is effective in 10 fields requiring more advanced levels of CONTACT ANGLE TO PURE WATER (°) material modification. 0 PET TAC PPS PVA COP Acrylic Polyimide Glass epoxy Polyethylene Polypropylene Polycarbonate ■Surface modification of resin ■Bonding pre-processing (improve adhesiveness) Example: Polyphenylene sulfide (PPS) + polyolefin (PO) 80° 3 15° Improved 2 about 3 times 1 Before excimer light irradiation After excimer light irradiation BONDING STRENGTH 0 Before processing After processing by excimer light by excimer light TLS B0013EA PRINCIPLE 1 Excimer lamp 2 Vacuum UV light In air O3 O(1D) O3 O(1D) O3 (Wavelength: 172 nm) 1 O2 O3 + O( D) Reaction O3 O(1D) O3 O(1D) O3 H HHH HHHH OH O OH COOH CCCC Resin material CCCC CCCC HHHH HHHH HHHH Vacuum UV light at a wavelength Bonds in material surface are Imparts hydrophilicity to the material of 172 nm generates ozone and simultaneously broken up by surface since chemical reaction active oxygen in large quantities. -
ENTERED ATOM Instrument Corporation, Ct Al., § August 16, 2018 § David J
Case 4:12-cv-01811 Document 142 Filed in TXSD on 08/16/18 Page 1 of 6 UNffiD STATES DISTRICT COURT SOUTHERN DISTRICT UnitedOF TEXAS States District Court Southern District of Texas ENTERED ATOM Instrument Corporation, ct al., § August 16, 2018 § David J. Bradley, Clerk Plaintiffs, § § 1Jcrsus § Civil Action H-I2-r8II § § Petroleum Analyzer Company, L.P., § § Defendant. § Findings and Conclusions 1. Background. Franek Olstowski worked for Petroleum Analyzer Company, L.P., before becoming president and part-owner of ATOM Instrument Corporation. ATOM and Petroleum develop, manufacture, and repair instruments for chemical analysis of hydrocarbons. In 2002, while working as a consultant for Petroleum, Olstowski developed an excimer light source to detect sulfur using ultraviolet fluorescence. He did this separately from his work at Petroleum. In 2003 and 2005, under a non-disclosure agreement, Petroleum and he talked about licensing his technology but did not reach an agreement. Olstowski was awarded a patent in 2007. Excimer is short for excited dimer. It is a combination of a noble gas and a reactive gas that produces ultraviolet light when excited by electricity. Possible combinations include krypton and chloride, xenon and chloride, and xenon and bromine. An excimer detects, in this case, sulfur by making it glow. like an excimer, :z;inc or cadmium can be used as a source of ultraviolet light. In 2006, Petroleum sued ATOM and Olstowski in Texas state court, claiming ownership of the excimer technology. In their contract, Olstowski and Petroleum had agreed to arbitrate, so the court sent them to do that. The arbitration panel awarded Olstowski ownership of all the technology. -
Excimer Lamps/Excimer Irradiation Unit | USHIO INC
11/18/2020 Excimer lamps/Excimer irradiation unit | USHIO INC. HOME Products Information Products Excimer lamps/Excimer irradiation unit Excimer lamps/Excimer irradiation unit Modification Cleaning MEMS, Electronic Semiconductors Components Liquid Crystal Display Printed Circuit Board and Functional Materials Biology and Chemistry PKG Energy Printing Excimer VUV light is the very high-energy light generated by lamps containing noble gases or noble-gas hydride compounds. Externally applying high-energy electrons to a sealed lamp containing a noble gas or noble gas hydride compound generates intense plasma discharge (dielectric barrier discharge). This plasma features high-energy electron content, and can be extinguished instantly. The plasma discharge instantly excites the atoms of the discharge gas (noble gas) to their excimer (Xe) state (high- energy orbital atoms form excimer excited molecules). The excimer-specific spectrum is emitted when atoms return from this excimer state to their original condition (ground state). This emission is called VUV light. Download * * * * Favorites Print catalog For inquiries regarding "222 nm ultraviolet (UV-C) light antibacterial and viral inactivation device," please contact : Sales Department 5, Sales Division, Light Source Business Division TEL: +81-3-5657-1016 / E-Mail: [email protected] Features Main applications Case study Product Lineup Options FAQ High photon energy VUV (vacuum ultraviolet) light at a wavelength of 180 nm or less, which is not available using conventional UV lamps, emits light efficiently to evoke or accelerate chemical reactions otherwise unachievable with conventional UV light. Single wavelength Excimer emission features a single peak emission wavelength with light emitted only in a very narrow range around the peak. -
I. Excimer Fluorescence, II. Heavy-Atom Spin-Orbital Coupling Effect and Iii. the Electronic Spectra of Ferrocene." (1965)
Louisiana State University LSU Digital Commons LSU Historical Dissertations and Theses Graduate School 1965 Studies in Molecular Spectroscopy; I. Excimer Fluorescence, II. Heavy-Atom Spin-Orbital Coupling Effect and IIi. The lecE tronic Spectra of Ferrocene. Fred Jewel Smith Louisiana State University and Agricultural & Mechanical College Follow this and additional works at: https://digitalcommons.lsu.edu/gradschool_disstheses Recommended Citation Smith, Fred Jewel, "Studies in Molecular Spectroscopy; I. Excimer Fluorescence, II. Heavy-Atom Spin-Orbital Coupling Effect and IIi. The Electronic Spectra of Ferrocene." (1965). LSU Historical Dissertations and Theses. 1092. https://digitalcommons.lsu.edu/gradschool_disstheses/1092 This Dissertation is brought to you for free and open access by the Graduate School at LSU Digital Commons. It has been accepted for inclusion in LSU Historical Dissertations and Theses by an authorized administrator of LSU Digital Commons. For more information, please contact [email protected]. This dissertation has bssn microfilmed exactly as received 6 6 -7 4 8 SMITH, Fred Jewel, 1939- STUDIES IN MOLECULAR SPECTROSCOPY} I. EXCIMER FLUORESCENCE, II. HEAVY- ATOM SPIN-ORBITAL COUPLING EFFECT AND HI. THE ELECTRONIC SPECTRA OF FERROCENE. Louisiana State University, Ph.D., 1965 Chemistry, physical University Microfilms, Inc., Ann Arbor, Michigan STUDIES IN MOLECULAR SPECTROSCOPY; I. EXCIMER FLUORESCENCE, II. HEAVY-ATOM SPIN-ORBITAL COUPLING EFFECT AND III . THE ELECTRONIC SPECTRA OF FERROCENE A Dissertation Submitted to the Graduate Faculty of the Louisiana State University and Agricultural and Mechanical College in partial fulfillment of the requirements for the degree of Doctor of Philosophy in The Department of Chemistry by Fred Jewel Smith B.A., University of Southern Mississippi, 1960 August, 1965 ACKNOWLEDGMENT The author wishes to express his sincere appreciation to Dr. -
New Applications with the EX-Mini Compact Excimer Lamp Light Source
2015 01 INTERVIEW PAGE 6 New Applications with the EX-mini Compact Excimer Lamp Light Source OPTO-SEMICONDUCTOR PRODUCTS PAGE 15 ELECTRON TUBE PRODUCTS PAGE 19 SYSTEMS PRODUCTS PAGE 26 Low-noise MPPC for precision Deep UV light source – higher Easy-to-use – NanoZoomer-SQ measurement power than LED Digital Slide Scanner June 22-25, 2015 Munich, Germany Hall A2, Booth 303 PHOTONNOVATION Content Medical Life ScienceDrug DiscoveryMeasurementAnalytical Semicond. Prod.Optical CommsSecurity Industry ND InspectionAcademic Research OPTO-SEMICONDUCTOR PRODUCTS 15 MPPC®/MPPC Module S13360 Series, C13365/C13366 Series 16 CMOS Linear Image Sensor S13131 17 Mini-spectrometer C13053MA 18 InAsSb Photovoltaic Detector (Non-cooled Type) P13243 Series ELECTRON TUBE PRODUCTS 19 Deep UV Light Source (UVCL) L12848-305 20 Excimer Lamp Light Source L11751-01, E12499, C11997 21 Opto-Spectrum Generator L12194-00-34054 22 NIR-PMT Unit H12397-75 23 Fast Decay Time Phosphor J12782-09D SYSTEMS PRODUCTS 24 ORCA-Flash4.0 LT with W-VIEW Mode™ 26 NanoZoomer-SQ Digital Slide Scanner C13140-21 27 ImagEM X2-1K EM-CCD Camera C9100-24B LASER PRODUCTS 28 LD Irradiation Light Source (SPOLD) L11785-61 29 Super Luminescent Diode (SLD) L12856-04 CompaNY NEWS APPLICATION REPORT 4 Hamamatsu holds the IEEE Milestone dedication ceremony 10 Tumor detection in fluorescent tissue microarrays enables high-through- in recognition of 20-inch photomultiplier tubes put analysis of multiple cancer biomarkers 5 Hamamatsu establishes a new subsidiary to enhance sales 12 Investigations of emission -
6 5 • Vacuum Ultraviolet Ar Excimer Emission Initiated by High Intensity Laser Produced Electrons
JP0050803 JAERI-Cont" 2000-006 6 5 • Vacuum Ultraviolet Ar Excimer Emission Initiated by High Intensity Laser Produced Electrons Shoichi Kubodera and Wataru Sasaki Department of Electrical and Electronic Engineering and Photon Science Center, Miyazaki University Gakuen Kibanadai Nishi 1-1, Miyazaki, 889-2192 Japan We have observed Ar2* emission using a tabletop femtosecond high intensity laser as an excitation source. High intensity laser produced electrons via an optical field induced ionization (OFI) process initiated the Ar2* production kinetics, which made themselves analogous to those produced in an electron beam produced plasma. A fast conductive cooling of the OFI plasma was found to be appropriate to initiate the excimer formation kinetics more efficiently. Keywords: Vacuum ultraviolet, Excimer molecule, Short pulse laser, Conductive cooling There have been considerable demands for the development of compact short wavelength lasers in the vacuum ultraviolet (VUV) spectral region. Such compact short wavelength lasers would be applicable to various scientific and industrial fields, such as photochemistry, biological science, and new types of materials processing. Currently available practical compact VUV lasers are the ArF excimer laser at 193 tun and the F2 laser at 157 run, both of which are excited by a compact discharge device. Recently more attention is paid to short wavelength lasers in the VUV in the future optical lithography industry. Rare gas excimers have long been one of the very few laser media in the VUV spectral region [1]. The emission wavelength of Ar2* is 126 run which is long enough to use transmission optical elements such as MgF2 and LiF. The Kr2* laser has an even longer emission wavelength centered at 147 mn which relaxes the conditions for optics and would become a competitor to the F2 laser at 157 run. -
New Approaches in Optical Lithography Technology for Subwavelength Resolution
Rochester Institute of Technology RIT Scholar Works Theses 5-2005 New approaches in optical lithography technology for subwavelength resolution Hoyoung Kang Follow this and additional works at: https://scholarworks.rit.edu/theses Recommended Citation Kang, Hoyoung, "New approaches in optical lithography technology for subwavelength resolution" (2005). Thesis. Rochester Institute of Technology. Accessed from This Dissertation is brought to you for free and open access by RIT Scholar Works. It has been accepted for inclusion in Theses by an authorized administrator of RIT Scholar Works. For more information, please contact [email protected]. NEW APPROACHES IN OPTICAL LITHOGRAPHY TECHNOLOGY FOR SUBW A VELENGTH RESOLUTION by Hoyoung Kang M.S. Hanyang University (1987) A dissertation submitted in partial fulfillment of the requirements for the degree of Ph.D. in the Chester F. Carson Center for Imaging Science of the College of Science Rochester Institute of Technology May 2005 Author HoyoungKang Hoyoung Kang . Accepted by CHESTER F. CARLSON CENTER FOR IMAGING SCIENCE COLLEGE OF SCIENCE ROCHESTER INSTITUTE OF TECHNOLOGY ROCHESTER, NEW YORK CERTIFICATE OF APPROVAL Ph. D. DEGREE DISSERTATION The Ph.D. Degree Dissertation of Hoyoung Kang has been examined and approved by the dissertation committee as satisfactory for the dissertation requirement for the Ph.D. degree in Imaging Science Bruce W. Smith Dr. Bruce W. Smith, Thesis Advisor Zoran Ninkov Dr. Zoran Ninkov M. Kotlarchyk Dr. Michael Kotlarchyk Paul Michaloski Paul Michaloski, Date Thesis/Dissertation Author Permission Statement Title of thesis or dissertation: ________________---;- ____ N eu.l I'>.pproecb 1'0 OptIc 0..0 (!'t-b03k=A.phJ Te.cb'Y>C)lo~ y Nrumeofauthor: ____H_D~y~o_U_~ __ ~~ __~~~~~~~~ ___________~ ____ Degree: ph. -
STERILRAY Vs . LARSON ELECTRONICS
Case 3:21-cv-01166-X Document 1 Filed 05/21/21 Page 1 of 38 PageID 1 IN THE UNITED STATES DISTRICT COURT FOR THE NORTHERN DISTRICT OF TEXAS HIGH ENERGY OZONE LLC d/b/a FAR- ) UV STERILRAY and S. EDWARD ) NEISTER, ) ) Civil Action No. 3:21-cv-1166 Plaintiffs, ) ) JURY TRIAL DEMANDED v. ) ) LARSON ELECTRONICS LLC, ) ) Defendant. ) COMPLAINT Plaintiffs High Energy Ozone LLC d/b/a Far-UV Sterilray (“HEO3”) and Mr. S. Edward Neister (“Mr. Neister”) (collectively, “Plaintiffs”), allege as follows: INTRODUCTION 1. More than fifteen years ago, physicist S. Edward Neister developed and patented methods for deactivating or destroying harmful microorganisms using a new spectrum of ultraviolet (UV) light. Mr. Neister’s methods included the development and use of Krypton-Chloride excimer lamps that emit a peak wavelength at 222 nm in conjunction with other wavelengths. Unlike the 254 nm UV light—which had been used for decades for sanitization but was dangerous to humans—applying 222 nm UV light does not penetrate human skin or eyes, making it far better and more useful than traditional lamps and methods of use. 2. Mr. Neister’s patented technology became the foundation for the family business. Mr. Neister and his brother John Neister originally founded the company Case 3:21-cv-01166-X Document 1 Filed 05/21/21 Page 2 of 38 PageID 2 that would become HEO3 in 2005 in a small town in New Hampshire. HEO3 is producing and selling lamps designed to perform Mr. Neister’s patented methods of killing harmful microorganisms. 3. -
Targeted Phototherapy
Review TTargetedargeted pphototherapyhototherapy Article VVenkataramenkataram MysoreMysore ABSTRACT Centre for Advanced Phototherapy is one of the most important therapeutic modalities in dermatology. This fi eld has Dermatology, Bangalore, India seen several major advances in the recent years, the most recent being targeted phototherapy. Targeted phototherapy, which includes laser and nonlaser technologies, delivers light/laser AAddressddress forfor ccorrespondence:orrespondence: in the ultraviolet spectrum, of specifi c wavelength, specifi cally targeted at the affected skin Dr. Venkataram Mysore, Venkat Charmalaya - Centre and thereby avoids many of the side effects of conventional phototherapy. The treatment for Advanced Dermatology, has been claimed to be effective, quick, and needing fewer treatment sessions. The article 3437 1st G cross 7 main reviews this new mode of phototherapy. Subbanna Garden, Vijay Nagar, Bangalore – 560 040, Key words: Excimer laser, excimer light, phototherapy, psoriasis, targeted phototherapy, India. E-mail: [email protected] vitiligo DDOI:OI: 10.4103/0378-6323.48655 PMID: 19293497 IINTRODUCTIONNTRODUCTION etc. These machines have the following disadvantages: 1. Exposure of uninvolved areas Phototherapy is used for a wide variety of skin diseases. 2. Slow delivery system and lengthy treatment There has been considerable progress in cellular sessions and cutaneous photobiology leading to improved 3. Multiple and frequent visits to clinic understanding of different photodermatoses and their 4. Difficulty in treating certain areas (such as treatment. However, the developments in phototherapy genitalia, oral mucosa, ear, etc) have been comparatively slow, as reflected in a recent 5. Difficulty in treating children who may feel publication that “developments in phototherapy have intimidated by the large machines not kept pace with scientific progress, as has been the 6. -
Excimer Lamp Pumped by a Triggered Discharge
ENTE PER LE NUOVE TECNOLOGIE, L'ENERGIA E L'AMBIENTE Dipartimento Innovazione ET f\T- - 9k 13 EXCIMER LAMP PUMPED BY A TRIGGERED DISCHARGE G. BALDACCHINI, S. BOLLANTI, P. Dl LAZZARO, F. FLORA, G. GIORDANO, T. LETARDI, A. RENIERI, G. SCHINA Centro Ricerche Frascati, Roma G. CLEMENTI, F. MUZZI, C.E. ZHENG EL.EN., (Electronic Engineering), Firenze MASTER DISTRIBUTION OF THIS DOCUMENT IS UNLIMITED FOREIGN SALES PROHIBITED RT/INN/96/13 DISCLAIMER Portions of this document may be illegible in electronic image products. Images are produced from the best available original document ABSTRACT Radiation characteristics and discharge performances of an excimer lamp are described. The discharge of the HCl/Xe gas mixture at an atmospheric pres sure, occurring near the quartz tube wall, is initiated by a trigger wire. A maximum total UV energy of about 0.4 J in a (0.8-0.9) ps pulse, radiated from a 10 cm discharge length, is obtained with a total discharge input energy of 8 J. Excimer lamps are the preferred choice for medical and material processing ir radiations, when the monochromaticity orcoherence of U V light is not required, due to their low cost, reliability and easy mantainance. (DISCHARGE UV LAMP). RIASSUNTO In questo iavoro vengono illustrate le caratteristiche di eccitazione e di emissione radiativa di ima lampada ad eccimeri. La scarica di eccitazione e innescata da un filo ad alia lensione e si sviluppa vicino alia parete del tubo di quarzo in una miscela di gas HCl/Xe a pressione atmosferica. Con un’energsa d; 8 J depositata dalla scarica in una lampada lunga 10 cm, si ottiene un’cnergia massima irraggiata nell’ultravioletto di 0.4 J in un impulse di 0.8-0.9 ps di durata. -
Excimer Lamp Excimer Lamp
RFRF DISCHARGEDISCHARGE TYPETYPE EXCIMEREXCIMER LAMPLAMP L12431L12431 Reforming Cleaning Making it "PERFECT" with "LIGHT" ! Making changes with "LIGHT" ! The possibilities of light for "Reforming" and "CLEANING" ! Principle Application examples Features of the Hamamatsu excimer lamps Reforming ISurface reforming of PET plastic Excimer lamp 80° Uniformity irradiates a large area Hamamatsu 1 long flat lamps Surface reforming technology is utilized in a 15° by use of a long flat lamp In air (wavelength 172 nm) wide range of industrial fields. Material re- Uniformity characteristics (along short axis) forming using excimer lamps is considered O2 O3 + O(1D) Long flat lamps vs. cylindrical lamps precision reforming compared to ordinary 3 1 3 1 3 O O( D) O O( D) O H2OH2O techniques because it occurs via a chemical H2O H2O Before irradiation After irradiation reaction on the atomic or molecular level. HHHH 100 Plastic I Moreover, this is a clean reforming that does CCCC CCCC Bonding pre-processing (improve adhesiveness) 90 material not harm the substrate or generate dust parti- Example: PPS (polyphenylene sulfide) + PO (polyolefin) 80 HHHH HHHH Good uniformity since entire surface is cles and so is an effective technology in 3 in close proximity Vacuum ultraviolet light at a wave- Bonds in material surface are bro- 70 fields requiring more advanced levels of mate- length of 172 nm generates ozone ken simultaneously with ozone and rial reforming. Improved 60 and active oxygen in large quanti- active oxygen generation, and the 2 Cylindrical lamps from ties. resulting moisture evaporates. about other companies Surface reforming using excimer lamps can 3 times 50 be used to increase adhesiveness and improve 40 1 functionality of materials such as by giving Bonding strength 30 UV power meter: Hamamatsu H9535-172 hydrophilicity to them, making it useful in a Lamp to UV power meter distance: 5 mm 2 20 Pinhole is interposed between lamp and UV power meter wide range of fields and materials.