Uva-DARE (Digital Academic Repository)
Total Page:16
File Type:pdf, Size:1020Kb
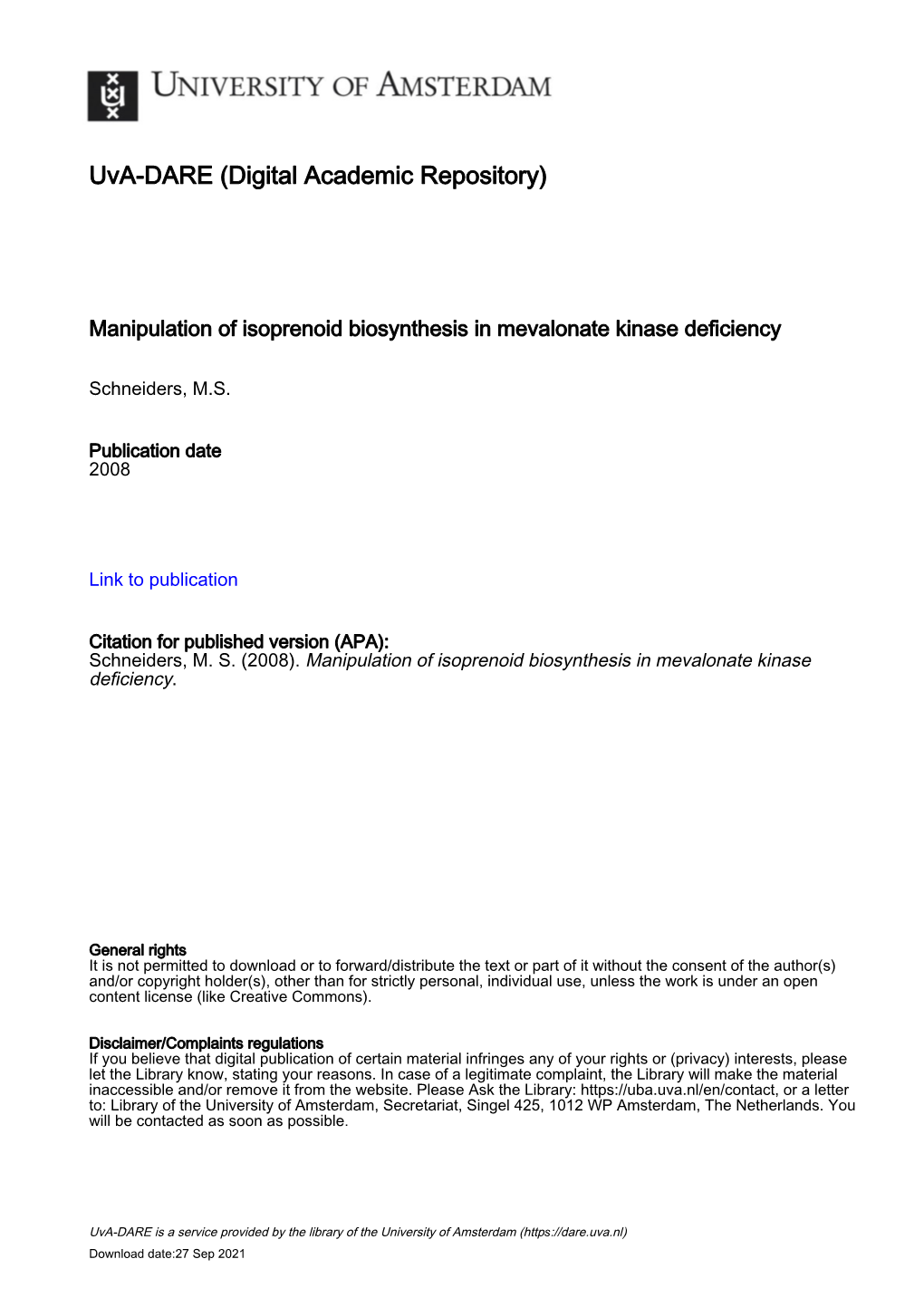
Load more
Recommended publications
-
PRODUCT INFORMATION Geranyl Pyrophosphate (Triammonium Salt) Item No
PRODUCT INFORMATION Geranyl Pyrophosphate (triammonium salt) Item No. 63320 CAS Registry No.: 116057-55-7 Formal Name: 3E,7-dimethyl-2,6-octadienyl- diphosphoric acid, triammonium salt Synonyms: GDP, Geranyl Diphosphate, GPP MF: C10H20O7P2 · 3NH3 FW: 365.3 O O Purity: ≥90% (NH +) – O P O P O Supplied as: A solution in methanol 4 3 Storage: -20°C O– O– Stability: ≥2 years Information represents the product specifications. Batch specific analytical results are provided on each certificate of analysis. Laboratory Procedures Geranyl pyrophosphate (triammonium salt) is supplied as a solution in methanol. To change the solvent, simply evaporate the methanol under a gentle stream of nitrogen and immediately add the solvent of choice. A stock solution may be made by dissoving the geranyl pyrophosphate (triammonium salt) in the solvent of choice. Geranyl pyrophosphate (triammonium salt) is slightly soluble in water. Description Geranyl pyrophosphate is an intermediate in the mevalonate pathway. It is formed from dimethylallyl pyrophosphate (DMAPP; Item No. 63180) and isopentenyl pyrophosphate by geranyl pyrophosphate synthase.1 Geranyl pyrophosphate is used in the biosynthesis of farnesyl pyrophosphate (Item No. 63250), geranylgeranyl pyrophosphate (Item No. 63330), cholesterol, terpenes, and terpenoids. Reference 1. Dorsey, J.K., Dorsey, J.A. and Porter, J.W. The purification and properties of pig liver geranyl pyrophosphate synthetase. J. Biol. Chem. 241(22), 5353-5360 (1966). WARNING CAYMAN CHEMICAL THIS PRODUCT IS FOR RESEARCH ONLY - NOT FOR HUMAN OR VETERINARY DIAGNOSTIC OR THERAPEUTIC USE. 1180 EAST ELLSWORTH RD SAFETY DATA ANN ARBOR, MI 48108 · USA This material should be considered hazardous until further information becomes available. -
Lanosterol 14Α-Demethylase (CYP51)
463 Lanosterol 14-demethylase (CYP51), NADPH–cytochrome P450 reductase and squalene synthase in spermatogenesis: late spermatids of the rat express proteins needed to synthesize follicular fluid meiosis activating sterol G Majdicˇ, M Parvinen1, A Bellamine2, H J Harwood Jr3, WWKu3, M R Waterman2 and D Rozman4 Veterinary Faculty, Clinic of Reproduction, Cesta v Mestni log 47a, 1000 Ljubljana, Slovenia 1Institute of Biomedicine, Department of Anatomy, University of Turku, Kiinamyllynkatu 10, FIN-20520 Turku, Finland 2Department of Biochemistry, Vanderbilt University School of Medicine, Nashville, Tennessee 37232–0146, USA 3Pfizer Central Research, Department of Metabolic Diseases, Box No. 0438, Eastern Point Road, Groton, Connecticut 06340, USA 4Institute of Biochemistry, Medical Center for Molecular Biology, Medical Faculty University of Ljubljana, Vrazov trg 2, SI-1000 Ljubljana, Slovenia (Requests for offprints should be addressed to D Rozman; Email: [email protected]) (G Majdicˇ is now at Department of Internal Medicine, UT Southwestern Medical Center, Dallas, Texas 75235–8857, USA) Abstract Lanosterol 14-demethylase (CYP51) is a cytochrome detected in step 3–19 spermatids, with large amounts in P450 enzyme involved primarily in cholesterol biosynthe- the cytoplasm/residual bodies of step 19 spermatids, where sis. CYP51 in the presence of NADPH–cytochrome P450 P450 reductase was also observed. Squalene synthase was reductase converts lanosterol to follicular fluid meiosis immunodetected in step 2–15 spermatids of the rat, activating sterol (FF-MAS), an intermediate of cholesterol indicating that squalene synthase and CYP51 proteins are biosynthesis which accumulates in gonads and has an not equally expressed in same stages of spermatogenesis. additional function as oocyte meiosis-activating substance. -
• Our Bodies Make All the Cholesterol We Need. • 85 % of Our Blood
• Our bodies make all the cholesterol we need. • 85 % of our blood cholesterol level is endogenous • 15 % = dietary from meat, poultry, fish, seafood and dairy products. • It's possible for some people to eat foods high in cholesterol and still have low blood cholesterol levels. • Likewise, it's possible to eat foods low in cholesterol and have a high blood cholesterol level SYNTHESIS OF CHOLESTEROL • LOCATION • All tissues • Liver • Cortex of adrenal gland • Gonads • Smooth endoplasmic reticulum Cholesterol biosynthesis and degradation • Diet: only found in animal fat • Biosynthesis: primarily synthesized in the liver from acetyl-coA; biosynthesis is inhibited by LDL uptake • Degradation: only occurs in the liver • Cholesterol is only synthesized by animals • Although de novo synthesis of cholesterol occurs in/ by almost all tissues in humans, the capacity is greatest in liver, intestine, adrenal cortex, and reproductive tissues, including ovaries, testes, and placenta. • Most de novo synthesis occurs in the liver, where cholesterol is synthesized from acetyl-CoA in the cytoplasm. • Biosynthesis in the liver accounts for approximately 10%, and in the intestines approximately 15%, of the amount produced each day. • Since cholesterol is not synthesized in plants; vegetables & fruits play a major role in low cholesterol diets. • As previously mentioned, cholesterol biosynthesis is necessary for membrane synthesis, and as a precursor for steroid synthesis including steroid hormone and vitamin D production, and bile acid synthesis, in the liver. • Slightly less than half of the cholesterol in the body derives from biosynthesis de novo. • Most cells derive their cholesterol from LDL or HDL, but some cholesterol may be synthesize: de novo. -
Cholesterol Metabolites 25-Hydroxycholesterol and 25-Hydroxycholesterol 3-Sulfate Are Potent Paired Regulators: from Discovery to Clinical Usage
H OH metabolites OH Review Cholesterol Metabolites 25-Hydroxycholesterol and 25-Hydroxycholesterol 3-Sulfate Are Potent Paired Regulators: From Discovery to Clinical Usage Yaping Wang 1, Xiaobo Li 2 and Shunlin Ren 1,* 1 Department of Internal Medicine, McGuire Veterans Affairs Medical Center, Virginia Commonwealth University, Richmond, VA 23249, USA; [email protected] 2 Department of Physiology and Pathophysiology, School of Basic Medical Sciences, Fudan University, Shanghai 200032, China; [email protected] * Correspondence: [email protected]; Tel.: +1-(804)-675-5000 (ext. 4973) Abstract: Oxysterols have long been believed to be ligands of nuclear receptors such as liver × recep- tor (LXR), and they play an important role in lipid homeostasis and in the immune system, where they are involved in both transcriptional and posttranscriptional mechanisms. However, they are increas- ingly associated with a wide variety of other, sometimes surprising, cell functions. Oxysterols have also been implicated in several diseases such as metabolic syndrome. Oxysterols can be sulfated, and the sulfated oxysterols act in different directions: they decrease lipid biosynthesis, suppress inflammatory responses, and promote cell survival. Our recent reports have shown that oxysterol and oxysterol sulfates are paired epigenetic regulators, agonists, and antagonists of DNA methyl- transferases, indicating that their function of global regulation is through epigenetic modification. In this review, we explore our latest research of 25-hydroxycholesterol and 25-hydroxycholesterol 3-sulfate in a novel regulatory mechanism and evaluate the current evidence for these roles. Citation: Wang, Y.; Li, X.; Ren, S. Keywords: oxysterol sulfates; oxysterol sulfation; epigenetic regulators; 25-hydroxysterol; Cholesterol Metabolites 25-hydroxycholesterol 3-sulfate; 25-hydroxycholesterol 3,25-disulfate 25-Hydroxycholesterol and 25-Hydroxycholesterol 3-Sulfate Are Potent Paired Regulators: From Discovery to Clinical Usage. -
Download File
Combined Biosynthetic and Synthetic Production of Valuable Molecules: A Hybrid Approach to Vitamin E and Novel Ambroxan Derivatives Bertrand T. Adanve Submitted in partial fulfillment of the requirements for the degree of Doctor of Philosophy in the Graduate School of Arts and Sciences COLUMBIA UNIVERSITY 2015 © 2015 Bertrand T. Adanve All Rights Reserved ABSTRACT Combined Biosynthetic and Synthetic Production of Valuable Molecules: A Hybrid Approach to Vitamin E and Novel Ambroxan Derivatives Bertrand T. Adanve Chapter 1. Introduction Synthetic chemistry has played a pivotal role in the evolution of modern life. More recently, the emerging field of synthetic biology holds the promise to bring about a paradigm shift with designer microbes to renewably synthesize complex molecules in a fraction of the time and cost. Still, given nature’s virtuosity at stitching a staggering palette of carbon frameworks with ease and synthetic chemistry’s superior parsing powers to access a greater number of unnatural end products, a hybrid approach that leverages the respective strengths of the two fields could prove advantageous for the efficient production of valuable natural molecules and their analogs. To demonstrate this approach, from biosynthetic Z,E-farnesol, we produced a library of novel analogs of the commercially important amber fragrance Ambrox®, including the first synthetic patchouli scent. Likewise, we produced the valuable tocotrienols from yeast-produced geranylgeraniol in a single step, the first such process of its kind. The novel acid catalyst system that allowed for this unique regioselective cyclization holds promise as an asymmetric proton transfer tool and could open the door to facile asymmetric synthesis of vitamin E and other molecules. -
Genetic Deletion of Abcc6 Disturbs Cholesterol Homeostasis in Mice Bettina Ibold1, Janina Tiemann1, Isabel Faust1, Uta Ceglarek2, Julia Dittrich2, Theo G
www.nature.com/scientificreports OPEN Genetic deletion of Abcc6 disturbs cholesterol homeostasis in mice Bettina Ibold1, Janina Tiemann1, Isabel Faust1, Uta Ceglarek2, Julia Dittrich2, Theo G. M. F. Gorgels3,4, Arthur A. B. Bergen4,5, Olivier Vanakker6, Matthias Van Gils6, Cornelius Knabbe1 & Doris Hendig1* Genetic studies link adenosine triphosphate-binding cassette transporter C6 (ABCC6) mutations to pseudoxanthoma elasticum (PXE). ABCC6 sequence variations are correlated with altered HDL cholesterol levels and an elevated risk of coronary artery diseases. However, the role of ABCC6 in cholesterol homeostasis is not widely known. Here, we report reduced serum cholesterol and phytosterol levels in Abcc6-defcient mice, indicating an impaired sterol absorption. Ratios of cholesterol precursors to cholesterol were increased, confrmed by upregulation of hepatic 3-hydroxy-3-methylglutaryl coenzyme A reductase (Hmgcr) expression, suggesting activation of cholesterol biosynthesis in Abcc6−/− mice. We found that cholesterol depletion was accompanied by a substantial decrease in HDL cholesterol mediated by lowered ApoA-I and ApoA-II protein levels and not by inhibited lecithin-cholesterol transferase activity. Additionally, higher proprotein convertase subtilisin/kexin type 9 (Pcsk9) serum levels in Abcc6−/− mice and PXE patients and elevated ApoB level in knockout mice were observed, suggesting a potentially altered very low-density lipoprotein synthesis. Our results underline the role of Abcc6 in cholesterol homeostasis and indicate impaired cholesterol metabolism as an important pathomechanism involved in PXE manifestation. Mutations in the adenosine triphosphate-binding cassette transporter C6 (ABCC6) gene are responsible for pseudoxanthoma elasticum (PXE), a metabolic disease, hallmarked by a progressive elastic fber calcifcation of the skin, eyes and cardiovascular system. -
Natural Isoprenoids Are Able to Reduce Inflammation in a Mouse
0031-3998/08/6402-0177 Vol. 64, No. 2, 2008 PEDIATRIC RESEARCH Printed in U.S.A. Copyright © 2008 International Pediatric Research Foundation, Inc. Natural Isoprenoids are Able to Reduce Inflammation in a Mouse Model of Mevalonate Kinase Deficiency ANNALISA MARCUZZI, ALESSANDRA PONTILLO, LUIGINA DE LEO, ALBERTO TOMMASINI, GIULIANA DECORTI, TARCISIO NOT, AND ALESSANDRO VENTURA Department of Reproductive and Developmental Sciences [A.M., L.L., A.T., TN, A.V.], Department of Biomedical Sciences [G.D.], University of Trieste, 34137 Trieste, Italy; Paediatric Division [A.P., A.T., T.N., A.V.], Institute of Child Health IRCCS Burlo Garofolo, 34137 Trieste, Italy ABSTRACT: Mevalonate kinase deficiency (MKD) is a rare ataxia, cerebellar atrophy, psychomotor retardation, and may disorder characterized by recurrent inflammatory episodes and, in die in early childhood (1). most severe cases, by psychomotor delay. Defective synthesis of HIDS patients usually are treated with anti-inflammatory isoprenoids has been associated with the inflammatory phenotype drugs and in particular corticosteroids; thalidomide is also in these patients, but the molecular mechanisms involved are still used but its effect is limited (2). In most severe cases, patients poorly understood, and, so far, no specific therapy is available for may benefit from treatment with biologic agents such as this disorder. Drugs like aminobisphosphonates, which inhibit the etanercept and anakinra (1,3–5). No treatment has been mevalonate pathway causing a relative defect in isoprenoids proven effective in curing the neurological symptoms in se- synthesis, have been also associated to an inflammatory pheno- type. Recent data asserted that cell inflammation could be reversed vere cases of MKD. -
Hop Aroma and Hoppy Beer Flavor: Chemical Backgrounds and Analytical Tools—A Review
Journal of the American Society of Brewing Chemists The Science of Beer ISSN: 0361-0470 (Print) 1943-7854 (Online) Journal homepage: http://www.tandfonline.com/loi/ujbc20 Hop Aroma and Hoppy Beer Flavor: Chemical Backgrounds and Analytical Tools—A Review Nils Rettberg, Martin Biendl & Leif-Alexander Garbe To cite this article: Nils Rettberg, Martin Biendl & Leif-Alexander Garbe (2018) Hop Aroma and Hoppy Beer Flavor: Chemical Backgrounds and Analytical Tools—A Review , Journal of the American Society of Brewing Chemists, 76:1, 1-20 To link to this article: https://doi.org/10.1080/03610470.2017.1402574 Published online: 27 Feb 2018. Submit your article to this journal Article views: 1464 View Crossmark data Full Terms & Conditions of access and use can be found at http://www.tandfonline.com/action/journalInformation?journalCode=ujbc20 JOURNAL OF THE AMERICAN SOCIETY OF BREWING CHEMISTS 2018, VOL. 76, NO. 1, 1–20 https://doi.org/10.1080/03610470.2017.1402574 Hop Aroma and Hoppy Beer Flavor: Chemical Backgrounds and Analytical Tools— A Review Nils Rettberga, Martin Biendlb, and Leif-Alexander Garbec aVersuchs– und Lehranstalt fur€ Brauerei in Berlin (VLB) e.V., Research Institute for Beer and Beverage Analysis, Berlin, Deutschland/Germany; bHHV Hallertauer Hopfenveredelungsgesellschaft m.b.H., Mainburg, Germany; cHochschule Neubrandenburg, Fachbereich Agrarwirtschaft und Lebensmittelwissenschaften, Neubrandenburg, Germany ABSTRACT KEYWORDS Hops are the most complex and costly raw material used in brewing. Their chemical composition depends Aroma; analysis; beer flavor; on genetically controlled factors that essentially distinguish hop varieties and is influenced by environmental gas chromatography; hops factors and post-harvest processing. The volatile fingerprint of hopped beer relates to the quantity and quality of the hop dosage and timing of hop addition, as well as the overall brewing technology applied. -
A Defective Response to Hedgehog Signaling in Disorders of Cholesterol Biosynthesis
letter A defective response to Hedgehog signaling in disorders of cholesterol biosynthesis Michael K. Cooper1,2,5, Christopher A. Wassif3, Patrycja A. Krakowiak3, Jussi Taipale1, Ruoyu Gong1, Richard I. Kelley4, Forbes D. Porter3 & Philip A. Beachy1 Published online 24 March 2003; doi:10.1038/ng1134 Smith–Lemli–Opitz syndrome (SLOS), desmosterolosis and lath- additional role for cholesterol in Hh signal response was sug- osterolosis are human syndromes caused by defects in the final gested by the observation that cyclopamine and jervine, terato- stages of cholesterol biosynthesis. Many of the developmental genic plant alkaloids that block Hh signaling, also inhibit malformations in these syndromes occur in tissues and struc- cholesterol transport and synthesis2,3. But cyclopamine has since tures whose embryonic patterning depends on signaling by the been shown to specifically inhibit Hh signaling by binding to a Hedgehog (Hh) family of secreted proteins. Here we report that pathway component4, and the doses of these alkaloids required response to the Hh signal is compromised in mutant cells from to inhibit Hh signaling are lower than those required to block mouse models of SLOS and lathosterolosis and in normal cells cholesterol transport (ref. 5 and M.K.C., unpublished data). pharmacologically depleted of sterols. We show that decreas- To determine how cholesterol may affect Hh signaling in embry- ing levels of cellular sterols correlate with diminishing respon- onic development, we exposed chick embryos to cyclodextrin, a http://www.nature.com/naturegenetics siveness to the Hh signal. This diminished response occurs at cyclic oligosaccharide that forms non-covalent complexes with sterol levels sufficient for normal autoprocessing of Hh protein, sterols6 and can be used to extract and deplete cholesterol from liv- which requires cholesterol as cofactor and covalent adduct. -
Table S1. Disease Classification and Disease-Reaction Association
Table S1. Disease classification and disease-reaction association Disorder class Associated reactions cross Disease Ref[Goh check et al. -
Mevalonate Kinase Deficiency: Therapeutic Targets, Treatments, and Outcomes
Expert Opinion on Orphan Drugs ISSN: (Print) 2167-8707 (Online) Journal homepage: http://www.tandfonline.com/loi/ieod20 Mevalonate kinase deficiency: therapeutic targets, treatments, and outcomes Annalisa Marcuzzi, Elisa Piscianz, Liza Vecchi Brumatti & Alberto Tommasini To cite this article: Annalisa Marcuzzi, Elisa Piscianz, Liza Vecchi Brumatti & Alberto Tommasini (2017): Mevalonate kinase deficiency: therapeutic targets, treatments, and outcomes, Expert Opinion on Orphan Drugs, DOI: 10.1080/21678707.2017.1328308 To link to this article: http://dx.doi.org/10.1080/21678707.2017.1328308 Accepted author version posted online: 08 May 2017. Submit your article to this journal View related articles View Crossmark data Full Terms & Conditions of access and use can be found at http://www.tandfonline.com/action/journalInformation?journalCode=ieod20 Download by: [The UC San Diego Library] Date: 12 May 2017, At: 02:40 Publisher: Taylor & Francis Journal: Expert Opinion on Orphan Drugs DOI: 10.1080/21678707.2017.1328308 Mevalonate kinase deficiency: therapeutic targets, treatments, and outcomes Annalisa Marcuzzi1, Piscianz Elisa1, Liza Vecchi Brumatti2, Alberto Tommasini2 1 Department of Medicine, Surgery, and Health Sciences, University of Trieste, Strada di Fiume, 447 Trieste, Italy. 34128 Trieste, Italy; 2 Institute for Maternal and Child Health – IRCCS "Burlo Garofolo", via dell’Istria, 65/1, 34137 Trieste, Italy. Annalisa Marcuzzi: BSc, PhD, Piscianz Elisa: BSc, PhD, Liza Vecchi Brumatti: MSc Alberto Tommasini: MD, PhD, Author to whom correspondence should be addressed: E-Mail: [email protected] Tel.: +39 040 3785422. Article highlights. • Mevalonate Kinase Deficiency (MKD) is a neglected disease with onset in infancy. • MKD clinical picture is characterized by recurrent fever attacks, abdominal pain arthralgia, lymphadenopathy and, in the most severe case, neurological involvement. -
Vitamin D Receptor Promotes Healthy Microbial Metabolites
www.nature.com/scientificreports OPEN Vitamin D receptor promotes healthy microbial metabolites and microbiome Ishita Chatterjee1, Rong Lu1, Yongguo Zhang1, Jilei Zhang1, Yang Dai 2, Yinglin Xia1 ✉ & Jun Sun 1 ✉ Microbiota derived metabolites act as chemical messengers that elicit a profound impact on host physiology. Vitamin D receptor (VDR) is a key genetic factor for shaping the host microbiome. However, it remains unclear how microbial metabolites are altered in the absence of VDR. We investigated metabolites from mice with tissue-specifc deletion of VDR in intestinal epithelial cells or myeloid cells. Conditional VDR deletion severely changed metabolites specifcally produced from carbohydrate, protein, lipid, and bile acid metabolism. Eighty-four out of 765 biochemicals were signifcantly altered due to the Vdr status, and 530 signifcant changes were due to the high-fat diet intervention. The impact of diet was more prominent due to loss of VDR as indicated by the diferences in metabolites generated from energy expenditure, tri-carboxylic acid cycle, tocopherol, polyamine metabolism, and bile acids. The efect of HFD was more pronounced in female mice after VDR deletion. Interestingly, the expression levels of farnesoid X receptor in liver and intestine were signifcantly increased after intestinal epithelial VDR deletion and were further increased by the high-fat diet. Our study highlights the gender diferences, tissue specifcity, and potential gut-liver-microbiome axis mediated by VDR that might trigger downstream metabolic disorders. Metabolites are the language between microbiome and host1. To understand how host factors modulate the microbiome and consequently alter molecular and physiological processes, we need to understand the metabo- lome — the collection of interacting metabolites from the microbiome and host.