Two-Higgs-Doublet Model with Soft CP-Violation Confronting Electric Dipole Moments and Colliders
Total Page:16
File Type:pdf, Size:1020Kb
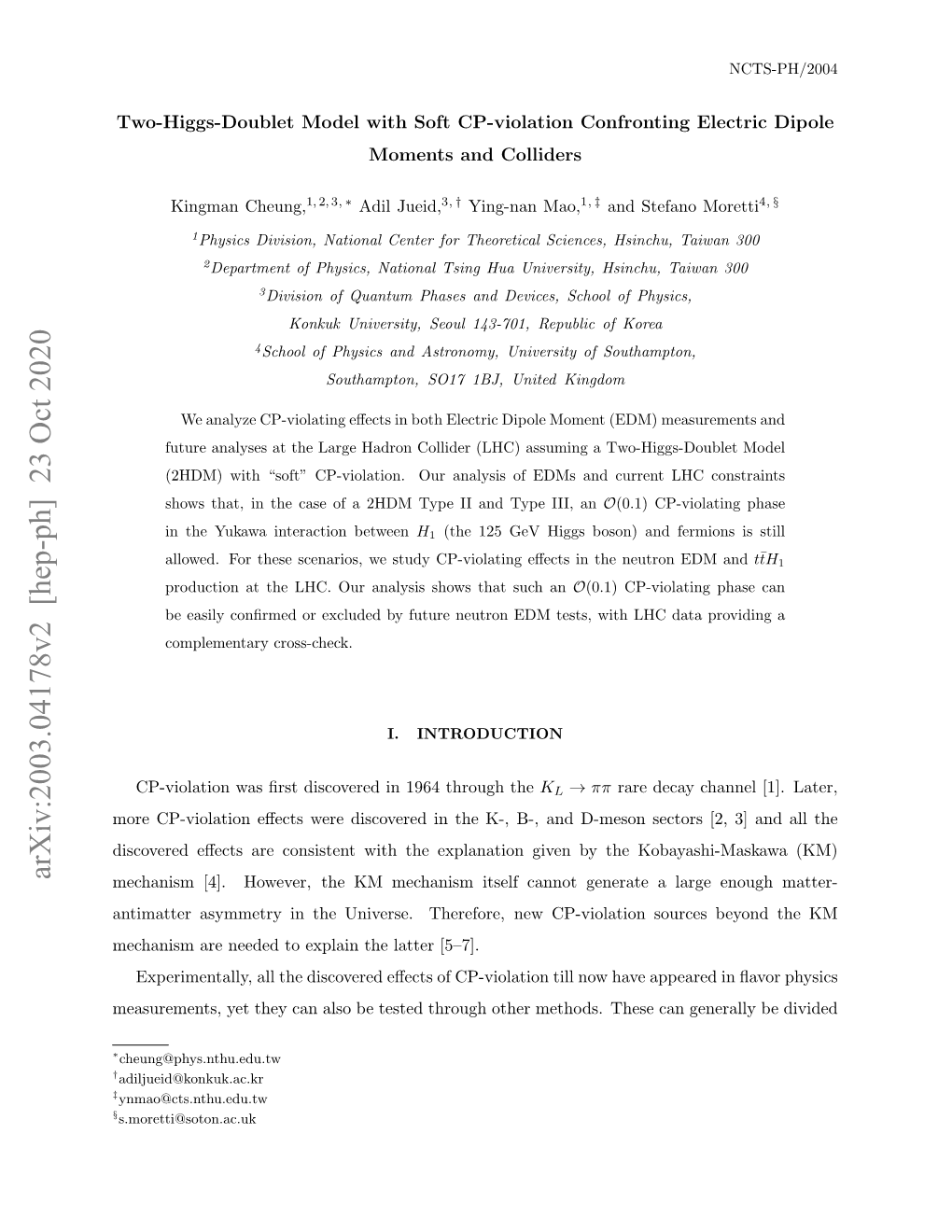
Load more
Recommended publications
-
Cryoedm: Building a Simulation Framework for Ultra-Cold Neutrons
CryoEDM: building a simulation framework for ultra-cold neutrons Matthew Rásó-Barnett University of Sussex • - CryoEDM • - What are UCN? • - Simulating UCN in CryoEDM Matthew Rásó-Barnett - University of Sussex - 6th April 2010 1 Wednesday, 6 April 2011 Why are we interested in EDMs anyway? • The existence of a permanent EDM in fundamental particles would be a violation of Time reversal symmetry and therefore CP symmetry. • CP violation is thought to be one of the key ingredients necessary for the predominance of matter over anti-matter in the universe. ➡ Sakharov’s necessary conditions for Baryogenesis • However, sources of CP-violation in the standard model are generally thought to be insufficient to generate presently observed baryon asymmetry. Matthew Rásó-Barnett - University of Sussex - 6th April 2010 2 Wednesday, 6 April 2011 Neutron EDM Measurements • Many different theoretical models predict new sources of CP-violation (such as the many from super- symmetry) and therefore give predictions for the size of the nEDM. • Thus, searches for a nEDM place experimental limits on many theories of beyond-standard-model physics Sussex-RAL 2006 Best Limit: Matthew Rásó-Barnett - University of Sussex - 6th April 2010 3 Wednesday, 6 April 2011 Measurement Principle • Measure changes in neutron’s Larmor precession frequency due to a potential EDM’s interaction with an applied Electric field. • Changing B field and E field from parallel to anti-parallel results in, !"#$%&'()"*+(,-.(,/0"%1+"'$ 2 S!"#*+',-#,+(.& polarizer analyzer incoming !" neutrons -
Pos(EPS-HEP 2009)376 , 1 Cm As Measured with E 26 − 10 × 9
Measuring the electric dipole moment of the neutron: The cryoEDM experiment 1 C A Baker, S N Balashov, V Francis, K Green, M G D van der Grinten, P S Iaydjiev , PoS(EPS-HEP 2009)376 S N Ivanov2, A Khazov3, M A H Tucker and D L Wark Science and Technology Facilities Council, Rutherford Appleton Laboratory, Chilton, Didcot OX11 0QX, UK. A Davidson, J R Grozier, M Hardiman, P G Harris, J R Karamath, K Katsika, J M Pendlebury, S J M Peeters, D B Shiers, P N Smith, C M Townsley and I Wardell University of Sussex, Falmer, Brighton BN1 9QH, UK. C Clarke∗4, S Henry, H Kraus and M McCann5 University of Oxford, Keble Road, Oxford OX1 3RH, UK. P Geltenbort Institut Laue-Langevin, BP156, F-38042 Grenoble, France. H Yoshiki University of Kure, Japan. 1on leave of absence from INRNE, Sofia, Bulgaria 2on leave of absence from PNPI, St Petersburg, Russia 3joint with the University of Sussex, Falmer, Brighton BN1 9QH, UK 4E-mail: [email protected] 5joint with Rutherford Appleton Laboratory, Chilton, Didcot, OXON OX11 0QX, UK The cryoEDM experiment at the Institut Laue-Langevin in Grenoble will measure the electric dipole moment (EDM) of the neutron with unparalleled precision. A neutron EDM arises due to CP violation. The cryoEDM experiment is sensitive to levels of CP violation predicted by many “beyond the standard model” theories and the result will therefore constrain or support these −26 theories. The current limit to the neutron EDM stands at jdnj < 2:9×10 e cm as measured with a room temperature experiment. -
Experimental Constraint on Quark Electric Dipole Moments
Experimental constraint on quark electric dipole moments Tianbo Liu,1, 2, ∗ Zhiwen Zhao,1 and Haiyan Gao1, 2 1Department of Physics, Duke University and Triangle Universities Nuclear Laboratory, Durham, North Carolina 27708, USA 2Duke Kunshan University, Kunshan, Jiangsu 215316, China The electric dipole moments (EDMs) of nucleons are sensitive probes of additional CP violation sources beyond the standard model to account for the baryon number asymmetry of the universe. As a fundamental quantity of the nucleon structure, tensor charge is also a bridge that relates nucleon EDMs to quark EDMs. With a combination of nucleon EDM measurements and tensor charge extractions, we investigate the experimental constraint on quark EDMs, and its sensitivity to CP violation sources from new physics beyond the electroweak scale. We obtain the current limits on quark EDMs as 1:27×10−24 e·cm for the up quark and 1:17×10−24 e·cm for the down quark at the scale of 4 GeV2. We also study the impact of future nucleon EDM and tensor charge measurements, and show that upcoming new experiments will improve the constraint on quark EDMs by about three orders of magnitude leading to a much more sensitive probe of new physics models. I. INTRODUCTION one can derive it from nucleon EDM measurements. A bridge that relates the quark EDM and the nucleon EDM Symmetries play a central role in physics. Discrete is the tensor charge, which is a fundamental QCD quan- symmetries, charge conjugate (C), parity (P), and time- tity defined by the matrix element of the tensor cur- reversal (T ), were believed to be conserved until the dis- rent. -
Coversheet for Thesis in Sussex Research Online
A University of Sussex MPhil thesis Available online via Sussex Research Online: http://sro.sussex.ac.uk/ This thesis is protected by copyright which belongs to the author. This thesis cannot be reproduced or quoted extensively from without first obtaining permission in writing from the Author The content must not be changed in any way or sold commercially in any format or medium without the formal permission of the Author When referring to this work, full bibliographic details including the author, title, awarding institution and date of the thesis must be given Please visit Sussex Research Online for more information and further details A New Monte-Carlo Based Simulation for the CryoEDM Experiment Matthew R´as´o-Barnett Submitted for the degree of Master of Philosophy School of Mathematical and Physical Sciences University of Sussex September 2013 Supervisors: Dr S J M Peeters and Prof P G Harris Declaration I hereby declare that this thesis describes my own original work, ex- cept where explicitly stated. No part of this work has previously been submitted, either in the same or different form, to this or any other University in connection with a higher degree or qualification. Matthew R´as´o-Barnett Signed: Date: March 26, 2015 2 University of Sussex Matthew R´as´o-Barnett,Master of Philosophy A new Monte-Carlo based simulation for the CryoEDM Experiment Abstract This thesis presents a new Monte-Carlo based simulation of the physics of ultra-cold neutrons (UCN) in complex geometries and its applica- tion to the CryoEDM experiment. It includes a detailed description of the design and performance of this simulation along with its use in a project to study the magnetic depolarisation time of UCN within the apparatus due to magnetic impurities in the measurement cell, which is a crucial parameter in the sensitivity of a neutron electric- dipole-moment (nEDM) experiment. -
Graduate School
xford hysics Post-Graduate Study Particle Physics We’ve discovered the Higgs so what else is there to do? 1 Some events selected in the Higgs search Higgs → γγ left Higgs → Z Z* below ATLAS H->gg Cleanest channels for very low Mass Higgs, Needs: Good di-photon mass resolution Determination of primary vertex Good Photon Id. Last year g/Jet, g/p0 discrimination Need to understand backgrounds with high precision with Data This year Driven techniques QCD gg production g-Jet and Jet-Jet production combined H→γγ search Local significance 4.5σ at Higgs mass 126.5 GeV 3 H→γγ and H → Z Z* are the best channels to tie down the Higgs mass but to check that this is the Higgs predicted by the Standard Model we have to check that it couples to all channels as predicted Is it a Standard Model Higgs? It is consistent with SM Here is H → W W* with W’s decaying via lepton neutrino No mass peak but a consistent level of enhancement at 3.2σ Combined channels: H → γγ, ZZ*, WW*, bb, τ+τ- Higgs excluded MH 110 to 122.7 and 129 to 557 GeV at 95% CL An excess at MH=126.5 with local significance 5σ, where 4.6σ would be expected for a Standard Model Higgs Lots more work to do: Are the branching ratios as predicted for SM Higgs? Is it spin-0? Is there only ONE of them? The Higgs was not the only reason we built the LHC there are many other questions • Do forces unify at high energy? •Are there extra dimensions? Possibly where gravity enters but other forces do not. -
Electric Dipole Moment
The status of the search for a nEDM and the new UCN sources Andreas Knecht Paul Scherrer Institut & Universität Zürich Currently at: ETH Zürich Outline Neutron Electric Dipole Moment New UCN Sources nEDM Experiments: CryoEDM Experiment @ ILL nEDM Experiment @ SNS nEDM Experiment @ PSI Andreas Knecht The New, the Rare and the Beautiful, 6. – 8. January 2010, Zürich 2 The Baryon Asymmetry of the Universe Observed:* -10 nB/n γγγ = 6 x 10 SM expectation:** -18 nB/n γγγ ~ 10 Sakharov 1967: B-violation C, CP-violation * WMAP data: Astrophys. J. Supp. 170 , 377 (2007) thermal non-equilibrium ** Riotto and Trodden: JETPAndreas Lett. Knecht 5, 24 (1967) The New, the Rare and the Beautiful, 6. – 8. January 2010,Ann. Zürich Rev. Nucl. Part. Sc. 49 , 35 (1999)3 Electric Dipole Moment Non-zero, permanent EDM violates both parity P and time reversal T → Violates CP → Understand mechanism of CP violation Andreas Knecht The New, the Rare and the Beautiful, 6. – 8. January 2010, Zürich 4 nEDM History Current best limit: × -26 dn < 2.9 10 ecm PRL 97, 131801 (2006) Andreas Knecht The New, the Rare and the Beautiful, 6. – 8. January 2010, Zürich 5 Measurement Principle An EDM couples to an electric field as a MDM couples to a magnetic field: B E Measure EDM from the difference of precession frequencies for parallel/anti- parallel fields: Andreas Knecht The New, the Rare and the Beautiful, 6. – 8. January 2010, Zürich 6 Ultracold Neutrons Neutrons with kinetic energies of ~ 100 neV (~ 5 m/s) Interactions: Gravitational: V g = 100 neV/m Magnetic: V m = 60 neV/T Strong: V F up to 350 neV Weak: n → p + e + ν Andreas Knecht The New, the Rare and the Beautiful, 6. -
Status of Neutron Electric Dipole Moment (EDM)
Status of Neutron Electric Dipole Moment (EDM) Searches • Motivation for EDM searches • How to measure neutron’s EDM • Present limit and how to improve it • nEDM experiment at SNS – Technical challenges & progress – Construction/Funding challenges & status • Worldwide efforts for neutron EDM B. Filippone Particlegenesis 2014 6/24/14 Why Look for EDMs? • Existence of unique EDM implies violation of Time Reversal Invariance • Time Reversal Violation seen in K0–K0 system • May also be seen in early Universe - Matter-Antimatter asymmetry but the Standard Model effect is too small ! Particle EDM Zoo • Paramagnetic atoms and polar molecules are very sensitive to de • Diamagnetic atoms are sensitive~ to quark “chromo-”EDM (gluon+photon) = d q and Θ ~ QCD d q • Neutron and proton sensitive to dq , & ΘQCD Observation or lack thereof in one system does not predict results for other systems 3 Relative EDM Sensitivities System Dependence Present Future Limit (e-cm) (e-cm) -16 -26 -28 n dn~ (3x10 )qQCD + <3x10 10 1 ~ 1 ~ 0.7(dd 4 du ) 0.6e(dd 2 du ) 199 -16 -29 -29 Hg dHg~ (0.001x10 )qQCD - <3x10 10 (?) ~ ~ 0.006e(dd du ) Searches for a Neutron EDM • E.M. Purcell and N.F. Ramsey, Phys. Rev. 78, 807 (1950) – Neutron Scattering – Searching for Parity Violation – Pioneered Neutron Beam Magnetic Resonance nEDM Sensitivity “Moore’s Law” Trapped Ultra- Future neutron Cold Neutrons EDM - UCN 1950 2000 2010 6 How to Measure an EDM 1. Inject polarized particle E-field 2. Rotate spin by p/2 3. Flip E-field direction 4. Measure frequency shift B-field 2 B 2d E h With E = 50 kV/cm, B = 3 T Neutron EDM of 1 x 10-28 e-cm gives Must know B very well D 5 nHz, 90 Hz 7 Best Neutron Limit: ILL-Grenoble neutron EDM Experiment Harris et al. -
1 Nucleons, Nuclei, and Atoms
1 Nucleons, Nuclei, and Atoms 1.1 Overview Despite the success of the Standard Model in explaining so many subatomic physics phenomena, we recognize that the model is incomplete and must eventually give way to a more fundamental description of nature. We have discovered massive neutrinos and associated flavor violation, which require the introduction of new mass terms in the Standard Model. We have an excess of baryons over antibaryons in our universe, indicating baryon-number-violating interactions and likely new sources of CP violation. We know from the inventory of matter and energy in our universe that the portion associated with Standard Model physics is only about 5% of the total. The rest remains unidentified and quite mysterious. These “big questions” – the origin of matter, the nature of neutrino mass, the identification of dark matter and dark energy - have driven the two major thrusts of subatomic physics. One is the effort to probe ever shorter distance scales by advancing the energy frontier. Today that frontier in represented by CERN’s LHC. The alternative is to seek signals of the new physics in subtle violations of symmetry in our low-energy world – ultraweak interactions that might mediate lepton- or baryon-number violation, generate electric dipole moments, or lead to unexpected flavor physics. This second approach is the theme of this chapter: ultrasensitive techniques in atomic, nuclear, and particle physics that might reveal the nature of our “next Standard Model.” Like particle astrophysics and cosmology, the third leg of our efforts to find new physics, this second approach uses our world as a laboratory, and depends on precision to identify the new physics. -
Lattice Nuclear Physics Investigations of Fundamental Symmetries
Lattice Nuclear Physics Investigations of Fundamental Symmetries Advisor: Tom Luu | Begin of Work: November 2014 31. July 2015 | Christopher Körber | Forschungszentrum Jülich & University of Bonn ECT* Talent — Training in Advanced Low Energy Nuclear Theory Mitglied der Helmholtz-Gemeinschaft 1 Asymmetry of Matter and Antimatter Symmetry of baryonic matter and antimatter after beginning of universe? Pair annihilation and radiation Observed asymmetry of matter Images by TED-Ed original: What happened to antimatter? Rolf Landua Mitglied der Helmholtz-Gemeinschaft Christopher Körber — ECT* Talent — 31. July 2015 2 2 Need for Charge-Parity Violation Sakharov conditions for explaining asymmetry Processes violate Processes violate Charge Generation of baryon or lepton and Charge-Parity symmetry antisymmetry outside number conservation (Weak interactions violate both) of thermal equilibrium X ‘æ B + Y ‘æ X ‘æ B + Y N = N ‘æC-Symmetry B B¯ T-Symmetry‘æ ¯ ¯ ¯ CP:CP Phase space X ‘æ B + Y cancellations Y + B ‘æ X Weak Standard Model interactions (CKM matrix) not sufficient to explain amount of asymmetry Mitglied der Helmholtz-Gemeinschaft Christopher Körber — ECT* Talent — 31. July 2015 3 3 Hadronic Hints for CP Violations: Electric Dipole Moment Electric Dipole Moment as a CP violating quantity S˛ 3 d˛ := d r ˛r fl(˛r )=d S˛ d˛ ⁄ Charge distribution Total nucleus spin P Magnetic Dipole Moment ˛ ˛ ˛ ˛ H = −B · (µ S) − E · (d S) T ˛ ˛ ˛ ˛ = −(−B) · (µ (−S)) − (+E) · (d (−S)) EDM violates CP CPT Mitglied der Helmholtz-Gemeinschaft Christopher Körber -
Lawrence Berkeley National Laboratory Recent Work
Lawrence Berkeley National Laboratory Recent Work Title Discovering the New Standard Model: Fundamental Symmetries and Neutrinos Permalink https://escholarship.org/uc/item/1f96954g Authors Cianciolo, V Balantekin, AB Bernstein, A et al. Publication Date 2012-12-20 Peer reviewed eScholarship.org Powered by the California Digital Library University of California Discovering the New Standard Model: Fundamental Symmetries and Neutrinos Report of the Fundamental Symmetries and Neutrinos Workshop August 10-11, 2012 – Chicago, IL V. Cianciolo,1 A. B. Balantekin,2 A. Bernstein,3 V. Cirigliano,4 M.D. Cooper,4 D. J. Dean,1 S. R. Elliott,4 B. W. Filippone,5 S. J. Freedman,6,7,a G.L. Greene,8,1 K.M. Heeger,2 D. W. Hertzog,9 B. R. Holstein,10 P. Huffman,11,1 T. Ito,4 K. Kumar,10 Z.-T. Lu,12 J. S. Nico,13 G. D. Orebi Gann,6,7 K. Paschke,14 A. Piepke,15 B. Plaster,16 D. Pocanic,14 A. W. P. Poon,7 D. C. Radford,1 M. J. Ramsey-Musolf,2 R. G. H. Robertson,9 G. Savard,12 K. Scholberg,17 Y. Semertzidis,18 J. F. Wilkerson19,20,1 1 Physics Division, Oak Ridge National Laboratory, Oak Ridge, TN 2 Department of Physics, University of Wisconsin, Madison, WI 3 Physics Division, Lawrence Livermore National Laboratory, Livermore, CA 4 Physics Division, Los Alamos National Laboratory, Los Alamos, NM 5 Department of Physics, California Institute of Technology, Pasadena, CA 6 Physics Department, University of California at Berkeley, Berkeley, CA 7 Nuclear Science Division, Lawrence Berkeley National Laboratory, Berkeley, CA 8 Department of Physics and Astronomy, University -
Neutron Electric Dipole Moment Measurement: Simultaneous Spin Analysis and Preliminary Data Analysis V
Neutron Electric Dipole Moment measurement: simultaneous spin analysis and preliminary data analysis V. Helaine To cite this version: V. Helaine. Neutron Electric Dipole Moment measurement: simultaneous spin analysis and prelimi- nary data analysis. Nuclear Experiment [nucl-ex]. Université de Caen, 2014. English. tel-01063399 HAL Id: tel-01063399 https://tel.archives-ouvertes.fr/tel-01063399 Submitted on 12 Sep 2014 HAL is a multi-disciplinary open access L’archive ouverte pluridisciplinaire HAL, est archive for the deposit and dissemination of sci- destinée au dépôt et à la diffusion de documents entific research documents, whether they are pub- scientifiques de niveau recherche, publiés ou non, lished or not. The documents may come from émanant des établissements d’enseignement et de teaching and research institutions in France or recherche français ou étrangers, des laboratoires abroad, or from public or private research centers. publics ou privés. Universit´ede Caen Basse-Normandie U.F.R Sciences Ecole´ doctorale: SIMEM Th`ese de doctorat Pr´esent´ee et soutenue le 8 Septembre 2014 par Monsieur Victor H´elaine pour obtenir le Doctorat de l’Universit´ede Caen Basse-Normandie Sp´ecialit´e: Constituants El´ementaires´ et Physique Th´eorique Mesure du moment dipolaire ´electrique du neutron: analyse simultan´ee de spin et analyse pr´eliminaire de donn´ees. Directeur de th`ese : Monsieur Gilles Ban Jury: P. Harris - Pr.UniversityofSussex(UK) Rapporteur O. Zimmer - Pr. TUM, Institut Laue-Langevin (Grenoble) Rapporteur G. Ban - Pr.Ensicaen,LPC(Caen) Directeurdeth`ese K. Kirch - Pr. ETH Z¨urich, Paul Scherrer Institute (Suisse) Examinateur L. Serin - DirecteurdeRechercheLAL(Orsay) Pr´esidentdujury G. -
SQUID Magnetometry for the Cryoedm Experiment—Tests at LSBB
Journal of Instrumentation OPEN ACCESS Related content - A Simple Strong SQUID SQUID magnetometry for the cryoEDM Keiz Murata, Terumi Kawai, Yasuyuki Sato et al. experiment—Tests at LSBB - CryoEDM: a cryogenic experiment to measure the neutron Electric Dipole Moment To cite this article: S Henry et al 2008 JINST 3 P11003 C A Baker, S N Balashov, V Francis et al. - Magnetic state of Nb(l-7nm)/Cu30Ni70 (6nm) superlattices revealed by Polarized Neutron Reflectometry and SQUID magnetometry View the article online for updates and enhancements. Yu. Khaydukov, R. Morari, D. Lenk et al. Recent citations - A SQUID magnetometry system for a cryogenic neutron electric dipole moment experiment S. Henry et al - Characterisation of superconducting capillaries for magnetic shielding of twisted-wire pairs in a neutron electric dipole moment experiment S. Henry et al - Tracking geomagnetic fluctuations to picotesla accuracy using two superconducting quantum interference device vector magnetometers S. Henry et al This content was downloaded from IP address 137.138.152.64 on 08/08/2018 at 09:03 PUBLISHED BY IOP PUBLISHING AND SISSA RECEIVED: July 10, 2008 REVISED: October 16, 2008 ACCEPTED: October 29, 2008 PUBLISHED: November 14, 2008 SQUID magnetometry for the cryoEDM experiment 2008 JINST 3 P11003 — Tests at LSBB a a a a b S. Henry, ∗ H. Kraus, M. Malek, V.B. Mikhailik and G. Waysand aUniversity of Oxford, Department of Physics, Denys Wilkinson Building, Keble Road, Oxford, OX1 3RH, U.K. bLaboratoire Souterrain à Bas Bruit de Rustrel-Pays d’Apt (LSBB), Université de Nice Sophia-Antipolis, La Grande Combe, 84400 Rustrel, France E-mail: [email protected] ABSTRACT: High precision magnetometry is an essential requirement of the cryoEDM experiment at the Institut Laue-Langevin, Grenoble.