Phosphoproteomics Reveals New Insights Into the Role of Pkng During the Persistence of 2 Pathogenic Mycobacteria in Host Macrophages
Total Page:16
File Type:pdf, Size:1020Kb
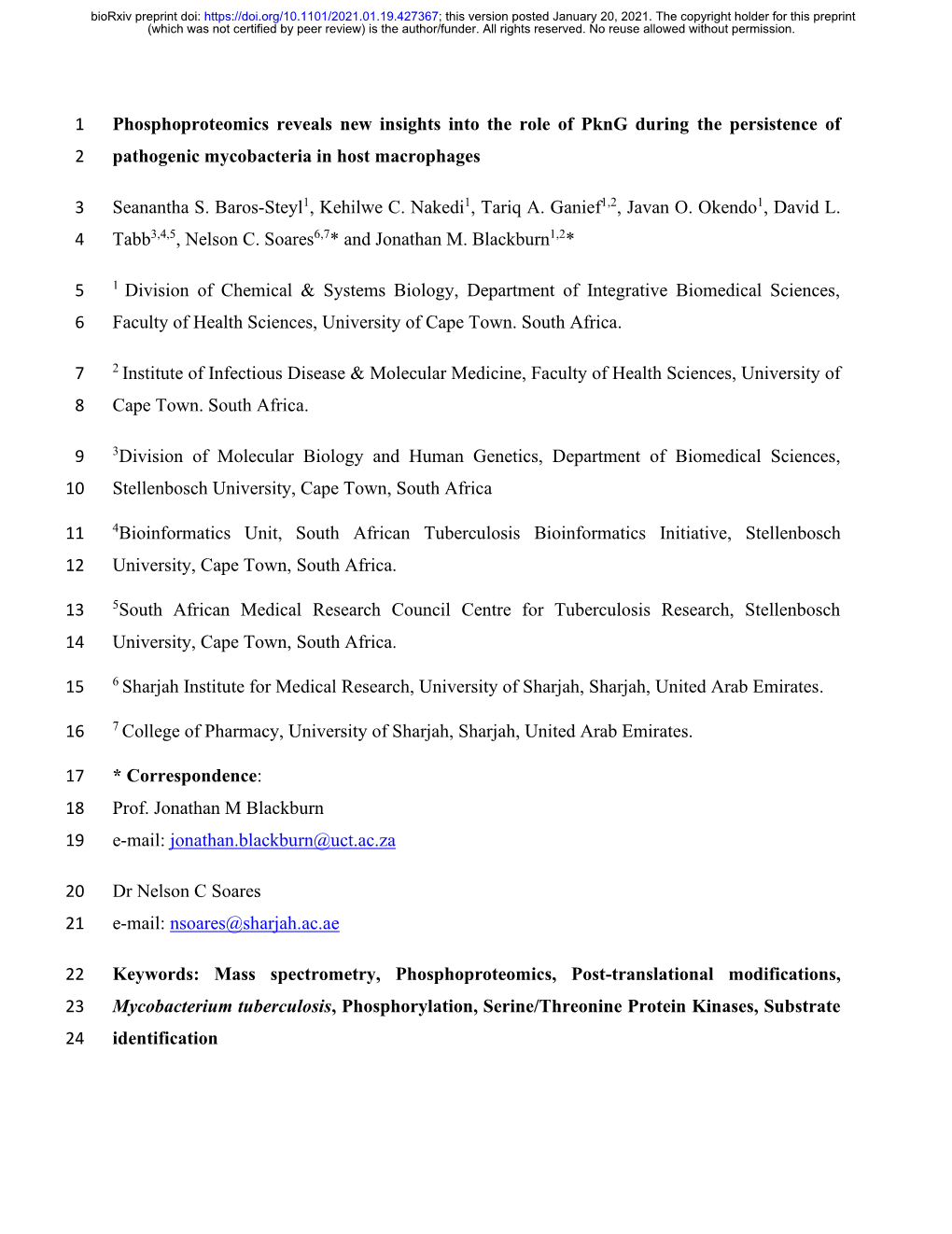
Load more
Recommended publications
-
Aberrant Methylation Underlies Insulin Gene Expression in Human Insulinoma
ARTICLE https://doi.org/10.1038/s41467-020-18839-1 OPEN Aberrant methylation underlies insulin gene expression in human insulinoma Esra Karakose1,6, Huan Wang 2,6, William Inabnet1, Rajesh V. Thakker 3, Steven Libutti4, Gustavo Fernandez-Ranvier 1, Hyunsuk Suh1, Mark Stevenson 3, Yayoi Kinoshita1, Michael Donovan1, Yevgeniy Antipin1,2, Yan Li5, Xiaoxiao Liu 5, Fulai Jin 5, Peng Wang 1, Andrew Uzilov 1,2, ✉ Carmen Argmann 1, Eric E. Schadt 1,2, Andrew F. Stewart 1,7 , Donald K. Scott 1,7 & Luca Lambertini 1,6 1234567890():,; Human insulinomas are rare, benign, slowly proliferating, insulin-producing beta cell tumors that provide a molecular “recipe” or “roadmap” for pathways that control human beta cell regeneration. An earlier study revealed abnormal methylation in the imprinted p15.5-p15.4 region of chromosome 11, known to be abnormally methylated in another disorder of expanded beta cell mass and function: the focal variant of congenital hyperinsulinism. Here, we compare deep DNA methylome sequencing on 19 human insulinomas, and five sets of normal beta cells. We find a remarkably consistent, abnormal methylation pattern in insu- linomas. The findings suggest that abnormal insulin (INS) promoter methylation and altered transcription factor expression create alternative drivers of INS expression, replacing cano- nical PDX1-driven beta cell specification with a pathological, looping, distal enhancer-based form of transcriptional regulation. Finally, NFaT transcription factors, rather than the cano- nical PDX1 enhancer complex, are predicted to drive INS transactivation. 1 From the Diabetes Obesity and Metabolism Institute, The Department of Surgery, The Department of Pathology, The Department of Genetics and Genomics Sciences and The Institute for Genomics and Multiscale Biology, The Icahn School of Medicine at Mount Sinai, New York, NY 10029, USA. -
Research Article Characterization, Tissue Expression, and Imprinting Analysis of the Porcine CDKN1C and NAP1L4 Genes
Hindawi Publishing Corporation Journal of Biomedicine and Biotechnology Volume 2012, Article ID 946527, 7 pages doi:10.1155/2012/946527 Research Article Characterization, Tissue Expression, and Imprinting Analysis of the Porcine CDKN1C and NAP1L4 Genes Shun Li,1 Juan Li,1 Jiawei Tian,1 Ranran Dong,1 Jin Wei,1 Xiaoyan Qiu,2 and Caode Jiang2 1 School of Life Science, Southwest University, Chongqing 400715, China 2 College of Animal Science and Technology, Southwest University, Chongqing 400715, China Correspondence should be addressed to Caode Jiang, [email protected] Received 4 August 2011; Revised 25 October 2011; Accepted 15 November 2011 Academic Editor: Andre Van Wijnen Copyright © 2012 Shun Li et al. This is an open access article distributed under the Creative Commons Attribution License, which permits unrestricted use, distribution, and reproduction in any medium, provided the original work is properly cited. CDKN1C and NAP1L4 in human CDKN1C/KCNQ1OT1 imprinted domain are two key candidate genes responsible for BWS (Beckwith-Wiedemann syndrome) and cancer. In order to increase understanding of these genes in pigs, their cDNAs are characterized in this paper. By the IMpRH panel, porcine CDKN1C and NAP1L4 genes were assigned to porcine chromosome 2, closely linked with IMpRH06175 and with LOD of 15.78 and 17.94, respectively. By real-time quantitative RT-PCR and polymorphism-based method, tissue and allelic expression of both genes were determined using F1 pigs of Rongchang and Landrace reciprocal crosses. The transcription levels of porcine CDKN1C and NAP1L4 were significantly higher in placenta than in other neonatal tissues (P<0.01) although both genes showed the highest expression levels in the lung and kidney of one- month pigs (P<0.01). -
Snps) Distant from Xenobiotic Response Elements Can Modulate Aryl Hydrocarbon Receptor Function: SNP-Dependent CYP1A1 Induction S
Supplemental material to this article can be found at: http://dmd.aspetjournals.org/content/suppl/2018/07/06/dmd.118.082164.DC1 1521-009X/46/9/1372–1381$35.00 https://doi.org/10.1124/dmd.118.082164 DRUG METABOLISM AND DISPOSITION Drug Metab Dispos 46:1372–1381, September 2018 Copyright ª 2018 by The American Society for Pharmacology and Experimental Therapeutics Single Nucleotide Polymorphisms (SNPs) Distant from Xenobiotic Response Elements Can Modulate Aryl Hydrocarbon Receptor Function: SNP-Dependent CYP1A1 Induction s Duan Liu, Sisi Qin, Balmiki Ray,1 Krishna R. Kalari, Liewei Wang, and Richard M. Weinshilboum Division of Clinical Pharmacology, Department of Molecular Pharmacology and Experimental Therapeutics (D.L., S.Q., B.R., L.W., R.M.W.) and Division of Biomedical Statistics and Informatics, Department of Health Sciences Research (K.R.K.), Mayo Clinic, Rochester, Minnesota Received April 22, 2018; accepted June 28, 2018 ABSTRACT Downloaded from CYP1A1 expression can be upregulated by the ligand-activated aryl fashion. LCLs with the AA genotype displayed significantly higher hydrocarbon receptor (AHR). Based on prior observations with AHR-XRE binding and CYP1A1 mRNA expression after 3MC estrogen receptors and estrogen response elements, we tested treatment than did those with the GG genotype. Electrophoretic the hypothesis that single-nucleotide polymorphisms (SNPs) map- mobility shift assay (EMSA) showed that oligonucleotides with the ping hundreds of base pairs (bp) from xenobiotic response elements AA genotype displayed higher LCL nuclear extract binding after (XREs) might influence AHR binding and subsequent gene expres- 3MC treatment than did those with the GG genotype, and mass dmd.aspetjournals.org sion. -
Letters to the Editor J Med Genet: First Published As 10.1136/Jmg.37.3.231 on 1 March 2000
210 Letters Letters to the Editor J Med Genet: first published as 10.1136/jmg.37.3.231 on 1 March 2000. Downloaded from J Med Genet 2000;37:210–212 No evidence of germline PTEN three reasons: somatic mutations have been found in PTEN in prostate tumours; germline mutations in Cowden mutations in familial prostate cancer disease produce a phenotype (although with no evidence of an associated susceptibility to prostate cancer); and PTEN deficient mice exhibit prostate abnormalities. We have therefore screened the Cancer Research Campaign/British EDITOR—Prostate cancer is the second most common Prostate Group (CRC/BPG) UK Familial Prostate Cancer cause of male cancer mortality in the UK.1 Current indica- Study samples for evidence of PTEN mutations. tions are that like many common cancers, prostate cancer The CRC/BPG UK Familial Prostate Cancer Study has has an inherited component.2 Segregation analysis has led collected lymphocyte DNA from 188 subjects from 50 to the proposed model of at least one highly penetrant, prostate cancer families. These families were chosen dominant gene (with an estimated 88% penetrance for because each contained three or more cases of prostate prostate cancer by the age of 85 in the highly susceptible cancer at any age or related sib pairs where at least one man population). Such a gene or genes would account for an was less than 67 (original criterion was 65) years old at estimated 43% of cases diagnosed at less than 55 years.2 diagnosis. In fact, the majority of the clusters consist of One prostate cancer susceptibility locus (HPC1) has been aVected sib pairs, with DNA often only available from reported on 1q24-253 and confirmed by Cooney et al4 and cases. -
RNA Splicing Regulated by RBFOX1 Is Essential for Cardiac Function in Zebrafish Karen S
© 2015. Published by The Company of Biologists Ltd | Journal of Cell Science (2015) 128, 3030-3040 doi:10.1242/jcs.166850 RESEARCH ARTICLE RNA splicing regulated by RBFOX1 is essential for cardiac function in zebrafish Karen S. Frese1,2,*, Benjamin Meder1,2,*, Andreas Keller3,4, Steffen Just5, Jan Haas1,2, Britta Vogel1,2, Simon Fischer1,2, Christina Backes4, Mark Matzas6, Doreen Köhler1, Vladimir Benes7, Hugo A. Katus1,2 and Wolfgang Rottbauer5,‡ ABSTRACT U2, U4, U5 and U6) and several hundred associated regulator Alternative splicing is one of the major mechanisms through which proteins (Wahl et al., 2009). Some of the best-characterized the proteomic and functional diversity of eukaryotes is achieved. splicing regulators include the serine-arginine (SR)-rich family, However, the complex nature of the splicing machinery, its associated heterogeneous nuclear ribonucleoproteins (hnRNPs) proteins, and splicing regulators and the functional implications of alternatively the Nova1 and Nova2, and the PTB and nPTB (also known as spliced transcripts are only poorly understood. Here, we investigated PTBP1 and PTBP2) families (David and Manley, 2008; Gabut et al., the functional role of the splicing regulator rbfox1 in vivo using the 2008; Li et al., 2007; Matlin et al., 2005). The diversity in splicing is zebrafish as a model system. We found that loss of rbfox1 led to further increased by the location and nucleotide sequence of pre- progressive cardiac contractile dysfunction and heart failure. By using mRNA enhancer and silencer motifs that either promote or inhibit deep-transcriptome sequencing and quantitative real-time PCR, we splicing by the different regulators. Adding to this complexity, show that depletion of rbfox1 in zebrafish results in an altered isoform regulating motifs are very common throughout the genome, but are expression of several crucial target genes, such as actn3a and hug. -
Mclean, Chelsea.Pdf
COMPUTATIONAL PREDICTION AND EXPERIMENTAL VALIDATION OF NOVEL MOUSE IMPRINTED GENES A Dissertation Presented to the Faculty of the Graduate School of Cornell University In Partial Fulfillment of the Requirements for the Degree of Doctor of Philosophy by Chelsea Marie McLean August 2009 © 2009 Chelsea Marie McLean COMPUTATIONAL PREDICTION AND EXPERIMENTAL VALIDATION OF NOVEL MOUSE IMPRINTED GENES Chelsea Marie McLean, Ph.D. Cornell University 2009 Epigenetic modifications, including DNA methylation and covalent modifications to histone tails, are major contributors to the regulation of gene expression. These changes are reversible, yet can be stably inherited, and may last for multiple generations without change to the underlying DNA sequence. Genomic imprinting results in expression from one of the two parental alleles and is one example of epigenetic control of gene expression. So far, 60 to 100 imprinted genes have been identified in the human and mouse genomes, respectively. Identification of additional imprinted genes has become increasingly important with the realization that imprinting defects are associated with complex disorders ranging from obesity to diabetes and behavioral disorders. Despite the importance imprinted genes play in human health, few studies have undertaken genome-wide searches for new imprinted genes. These have used empirical approaches, with some success. However, computational prediction of novel imprinted genes has recently come to the forefront. I have developed generalized linear models using data on a variety of sequence and epigenetic features within a training set of known imprinted genes. The resulting models were used to predict novel imprinted genes in the mouse genome. After imposing a stringency threshold, I compiled an initial candidate list of 155 genes. -
Is Associated with Hypomethylation At
797 ORIGINAL ARTICLE J Med Genet: first published as 10.1136/jmg.40.11.797 on 19 November 2003. Downloaded from Silencing of CDKN1C (p57KIP2) is associated with hypomethylation at KvDMR1 in Beckwith–Wiedemann syndrome N Diaz-Meyer, C D Day, K Khatod, E R Maher, W Cooper, W Reik, C Junien, G Graham, E Algar, V M Der Kaloustian, M J Higgins ............................................................................................................................... J Med Genet 2003;40:797–801 Context: Beckwith–Wiedemann syndrome (BWS) arises by several genetic and epigenetic mechanisms affecting the balance of imprinted gene expression in chromosome 11p15.5. The most frequent alteration associated with BWS is the absence of methylation at the maternal allele of KvDMR1, an intronic CpG island within the KCNQ1 gene. Targeted deletion of KvDMR1 suggests that this locus is an imprinting control region (ICR) that regulates multiple genes in 11p15.5. Cell culture based enhancer blocking assays indicate that KvDMR1 may function as a methylation modulated chromatin insulator and/or silencer. See end of article for Objective: To determine the potential consequence of loss of methylation (LOM) at KvDMR1 in the authors’ affiliations ....................... development of BWS. Methods: The steady state levels of CDKN1C gene expression in fibroblast cells from normal individuals, Correspondence to: and from persons with BWS who have LOM at KvDMR1, was determined by both real time quantitative M J Higgins, Department of Cancer Genetics, polymerase chain reaction (qPCR) and ribonuclease protection assay (RPA). Methylation of the CDKN1C Roswell Park Cancer promoter region was assessed by Southern hybridisation using a methylation sensitive restriction Institute, Buffalo, NY endonuclease. 14263, USA; michael.higgins@ Results: Both qPCR and RPA clearly demonstrated a marked decrease (86–93%) in the expression level of roswellpark.org the CDKN1C gene in cells derived from patients with BWS, who had LOM at KvDMR1. -
Human Proteins That Interact with RNA/DNA Hybrids
Downloaded from genome.cshlp.org on October 4, 2021 - Published by Cold Spring Harbor Laboratory Press Resource Human proteins that interact with RNA/DNA hybrids Isabel X. Wang,1,2 Christopher Grunseich,3 Jennifer Fox,1,2 Joshua Burdick,1,2 Zhengwei Zhu,2,4 Niema Ravazian,1 Markus Hafner,5 and Vivian G. Cheung1,2,4 1Howard Hughes Medical Institute, Chevy Chase, Maryland 20815, USA; 2Life Sciences Institute, University of Michigan, Ann Arbor, Michigan 48109, USA; 3Neurogenetics Branch, National Institute of Neurological Disorders and Stroke, NIH, Bethesda, Maryland 20892, USA; 4Department of Pediatrics, University of Michigan, Ann Arbor, Michigan 48109, USA; 5Laboratory of Muscle Stem Cells and Gene Regulation, National Institute of Arthritis and Musculoskeletal and Skin Diseases, Bethesda, Maryland 20892, USA RNA/DNA hybrids form when RNA hybridizes with its template DNA generating a three-stranded structure known as the R-loop. Knowledge of how they form and resolve, as well as their functional roles, is limited. Here, by pull-down assays followed by mass spectrometry, we identified 803 proteins that bind to RNA/DNA hybrids. Because these proteins were identified using in vitro assays, we confirmed that they bind to R-loops in vivo. They include proteins that are involved in a variety of functions, including most steps of RNA processing. The proteins are enriched for K homology (KH) and helicase domains. Among them, more than 300 proteins preferred binding to hybrids than double-stranded DNA. These proteins serve as starting points for mechanistic studies to elucidate what RNA/DNA hybrids regulate and how they are regulated. -
Associated with Spherical Equivalent
Molecular Vision 2016; 22:783-796 <http://www.molvis.org/molvis/v22/783> © 2016 Molecular Vision Received 20 August 2015 | Accepted 12 July 2016 | Published 14 July 2016 Variation in PTCHD2, CRISP3, NAP1L4, FSCB, and AP3B2 associated with spherical equivalent Fei Chen,1 Priya Duggal,1 Barbara E.K. Klein,2 Kristine E. Lee,2 Barbara Truitt,3 Ronald Klein,2 Sudha K. Iyengar,3 Alison P. Klein1,4,5 1Department of Epidemiology, Johns Hopkins Bloomberg School of Public Health, Baltimore, MD; 2Department of Ophthalmology and Visual Sciences, University of Wisconsin School of Medicine and Public Health, Madison, WI; 3Department of Epidemiology and Biostatistics, Case Western Reserve University, Cleveland, OH; 4Department of Oncology, Sidney Kimmel Comprehensive Cancer Center at Johns Hopkins, Baltimore, MD; 5Department of Pathology, Johns Hopkins School of Medicine, Baltimore, MD Purpose: Ocular refraction is measured in spherical equivalent as the power of the external lens required to focus images on the retina. Myopia (nearsightedness) and hyperopia (farsightedness) are the most common refractive errors, and the leading causes of visual impairment and blindness in the world. The goal of this study is to identify rare and low-frequency variants that influence spherical equivalent. Methods: We conducted variant-level and gene-level quantitative trait association analyses for mean spherical equiva- lent, using data from 1,560 individuals in the Beaver Dam Eye Study. Genotyping was conducted using the Illumina exome array. We analyzed 34,976 single nucleotide variants and 11,571 autosomal genes across the genome, using single-variant tests as well as gene-based tests. Results: Spherical equivalent was significantly associated with five genes in gene-based analysis: PTCHD2 at 1p36.22 (p = 3.6 × 10−7), CRISP3 at 6p12.3 (p = 4.3 × 10−6), NAP1L4 at 11p15.5 (p = 3.6 × 10−6), FSCB at 14q21.2 (p = 1.5 × 10−7), and AP3B2 at 15q25.2 (p = 1.6 × 10−7). -
1 SUPPLEMENTAL DATA Figure S1. Poly I:C Induces IFN-Β Expression
SUPPLEMENTAL DATA Figure S1. Poly I:C induces IFN-β expression and signaling. Fibroblasts were incubated in media with or without Poly I:C for 24 h. RNA was isolated and processed for microarray analysis. Genes showing >2-fold up- or down-regulation compared to control fibroblasts were analyzed using Ingenuity Pathway Analysis Software (Red color, up-regulation; Green color, down-regulation). The transcripts with known gene identifiers (HUGO gene symbols) were entered into the Ingenuity Pathways Knowledge Base IPA 4.0. Each gene identifier mapped in the Ingenuity Pathways Knowledge Base was termed as a focus gene, which was overlaid into a global molecular network established from the information in the Ingenuity Pathways Knowledge Base. Each network contained a maximum of 35 focus genes. 1 Figure S2. The overlap of genes regulated by Poly I:C and by IFN. Bioinformatics analysis was conducted to generate a list of 2003 genes showing >2 fold up or down- regulation in fibroblasts treated with Poly I:C for 24 h. The overlap of this gene set with the 117 skin gene IFN Core Signature comprised of datasets of skin cells stimulated by IFN (Wong et al, 2012) was generated using Microsoft Excel. 2 Symbol Description polyIC 24h IFN 24h CXCL10 chemokine (C-X-C motif) ligand 10 129 7.14 CCL5 chemokine (C-C motif) ligand 5 118 1.12 CCL5 chemokine (C-C motif) ligand 5 115 1.01 OASL 2'-5'-oligoadenylate synthetase-like 83.3 9.52 CCL8 chemokine (C-C motif) ligand 8 78.5 3.25 IDO1 indoleamine 2,3-dioxygenase 1 76.3 3.5 IFI27 interferon, alpha-inducible -
The Detailed 3D Multi-Loop Aggregate/Rosette Chromatin Architecture and Functional Dynamic Organization of the Human and Mouse G
bioRxiv preprint doi: https://doi.org/10.1101/064642; this version posted August 15, 2016. The copyright holder for this preprint (which was not certified by peer review) is the author/funder. All rights reserved. No reuse allowed without permission. The Detailed 3D Multi-Loop Aggregate/Rosette Chromatin Architecture and Functional Dynamic Organization of the Human and Mouse Genomes Tobias A. Knoch1*, Malte Wachsmuth2, Nick Kepper1,4, Michael Lesnussa1, Anis Abuseiris1, A. M. Ali Imam1,3, Petros Kolovos1,3, Jessica Zuin5, Christel E. M. Kockx6, Rutger W. W. Brouwer6, Harmen J. G. van de Werken3, Wilfred F. J. van IJken6, Kerstin S. Wendt5, and Frank G. Grosveld3 1Biophysical Genomics, Dept. Cell Biology & Genetics, Erasmus MC, Wytemaweg 80, 3015 CN Rotterdam, The Netherlands 2Cell Biology & Biophysics Unit, European Molecular Biology Laboratory, Meyerhofstr. 1, 69117 Heidelberg, Germany 3Cell Biology, Dept. Cell Biology & Genetics, Erasmus MC, Dr. Molewaterplein 50, 3015 GE Rotterdam, The Netherlands 4Genome Organization & Function, BioQuant & German Cancer Research Center, Im Neuenheimer Feld 267, 69120 Heidelberg, Germany 5Cohesin in Chromatin Structure and Gene Regulation, Dept. Cell Biology & Genetics, Erasmus MC, Dr. Molewaterplein 50, 3015 GE Rotterdam, The Netherlands 6Center for Biomics, Dept. Cell Biology & Genetics, Erasmus MC, Dr. Molewaterplein 50, 3015 GE Rotterdam, The Netherlands * corresponding author: [email protected] Knoch et al. 1 bioRxiv preprint doi: https://doi.org/10.1101/064642; this version posted August 15, 2016. The copyright holder for this preprint (which was not certified by peer review) is the author/funder. All rights reserved. No reuse allowed without permission. Abstract: The dynamic three-dimensional chromatin architecture of genomes and its co- evolutionary connection to its function – the storage, expression, and replication of genetic information – is still one of the central issues in biology. -
RBM25 Sirna (H): Sc-92256
SANTA CRUZ BIOTECHNOLOGY, INC. RBM25 siRNA (h): sc-92256 BACKGROUND STORAGE AND RESUSPENSION RMB25 (RNA binding motif protein 25), also known as S164, RNPC7, RED120 Store lyophilized siRNA duplex at -20° C with desiccant. Stable for at least or fSAP94, is a 784 amino acid protein that contains one PWI domain and one one year from the date of shipment. Once resuspended, store at -20° C, RRM (RNA recognition motif) domain. Localized to the cytoplasm, as well as avoid contact with RNAses and repeated freeze thaw cycles. to nuclear speckles, RBM25 functions as a splicing factor that is thought to Resuspend lyophilized siRNA duplex in 330 µl of the RNAse-free water stabilize the pre-mRNA-U1 snRNP complex and may couple splicing with provided. Resuspension of the siRNA duplex in 330 µl of RNAse-free water mRNA 3'-end formation. The gene encoding RBM25 maps to human chromo- makes a 10 µM solution in a 10 µM Tris-HCl, pH 8.0, 20 mM NaCl, 1 mM some 14, which houses over 700 genes and comprises nearly 3.5% of the EDTA buffered solution. human genome. Chromosome 14 encodes the presinilin 1 (PSEN1) gene, which is one of the three key genes associated with the development of Alzheimer’s APPLICATIONS disease (AD). The SERPINA1 gene is also located on chromosome 14 and, when defective, leads to the genetic disorder a1-antitrypsin deficiency, which RBM25 siRNA (h) is recommended for the inhibition of RBM25 expression in is characterized by severe lung complications and liver dysfunction. human cells. REFERENCES SUPPORT REAGENTS 1.