Power Output of America's Cup Grinders Can Be Improved with a Biomechanical Technique Intervention
Total Page:16
File Type:pdf, Size:1020Kb
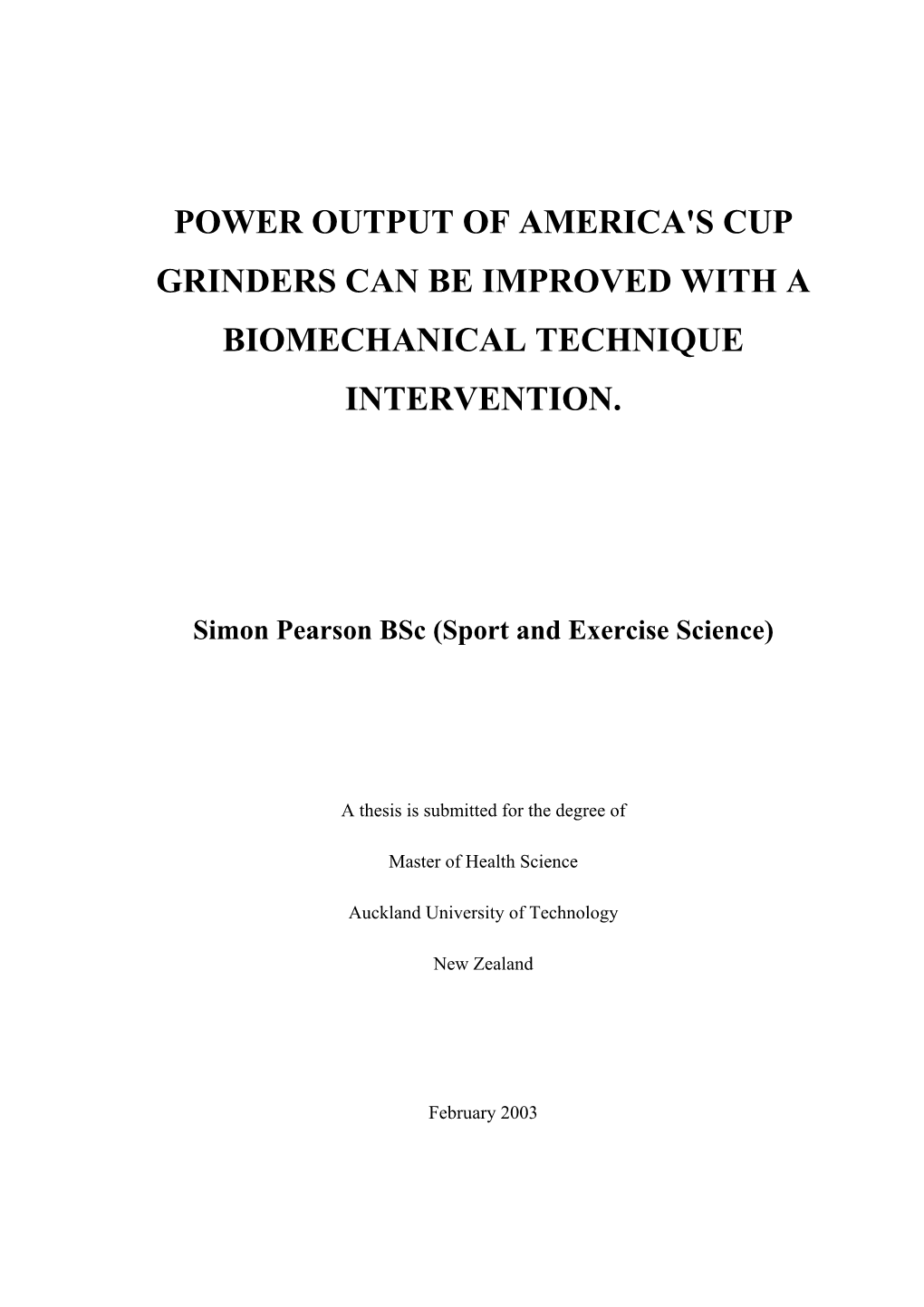
Load more
Recommended publications
-
Team Portraits Emirates Team New Zealand - Defender
TEAM PORTRAITS EMIRATES TEAM NEW ZEALAND - DEFENDER PETER BURLING - SKIPPER AND BLAIR TUKE - FLIGHT CONTROL NATIONALITY New Zealand HELMSMAN HOME TOWN Kerikeri NATIONALITY New Zealand AGE 31 HOME TOWN Tauranga HEIGHT 181cm AGE 29 WEIGHT 78kg HEIGHT 187cm WEIGHT 82kg CAREER HIGHLIGHTS − 2012 Olympics, London- Silver medal 49er CAREER HIGHLIGHTS − 2016 Olympics, Rio- Gold medal 49er − 2012 Olympics, London- Silver medal 49er − 6x 49er World Champions − 2016 Olympics, Rio- Gold medal 49er − America’s Cup winner 2017 with ETNZ − 6x 49er World Champions − 2nd- 2017/18 Volvo Ocean Race − America’s Cup winner 2017 with ETNZ − 2nd- 2014 A class World Champs − 3rd- 2018 A class World Champs PATHWAY TO AMERICA’S CUP Red Bull Youth America’s Cup winner with NZL Sailing Team and 49er Sailing pre 2013. PATHWAY TO AMERICA’S CUP Red Bull Youth America’s Cup winner with NZL AMERICA’S CUP CAREER Sailing Team and 49er Sailing pre 2013. Joined team in 2013. AMERICA’S CUP CAREER DEFINING MOMENT IN CAREER Joined ETNZ at the end of 2013 after the America’s Cup in San Francisco. Flight controller and Cyclor Olympic success. at the 35th America’s Cup in Bermuda. PEOPLE WHO HAVE INFLUENCED YOU DEFINING MOMENT IN CAREER Too hard to name one, and Kiwi excelling on the Silver medal at the 2012 Summer Olympics in world stage. London. PERSONAL INTERESTS PEOPLE WHO HAVE INFLUENCED YOU Diving, surfing , mountain biking, conservation, etc. Family, friends and anyone who pushes them- selves/the boundaries in their given field. INSTAGRAM PROFILE NAME @peteburling Especially Kiwis who represent NZ and excel on the world stage. -
Americas Cup Newsletter
AMERICAS CUP NEWSLETTER If you have problems to view this message click here. Official America's Cup Competitor Slate to be Revealed June 15 San Francisco, Calif., Wednesday, June 08, 2011 WHAT: The official competitors of the 34th America’s Cup will be formally welcomed by the Honorable Edwin M. Lee to San Francisco, site of the first two 2012 America’s Cup World Series events; and home of the 2013 Louis Vuitton Cup - America’s Cup Challenger Series - and America’s Cup Finals. WHEN: Wednesday, June 15th 10:30 A.M. PT WHO: Mayor Edwin M. Lee, City and County of San Francisco Iain Murray, CEO and Regatta Director, Americas Cup Race Management Richard Worth, Chairman, America’s Cup Event Authority Russell Coutts, CEO, ORACLE Racing Representatives of the Challengers for the 34th America’s Cup including Artemis Racing, China Team, Emirates Team New Zealand and others. WHERE: Ferry Building 2nd floor mezzanine San Francisco, Calif. NOTE: Members of the media are also invited to attend two events featuring the AC45 wing-sailed catamaran on Monday, June 13. Both events will held at Golden Gate Yacht Club, in the Marina, San Francisco. Monday, June 13 ● 10:00 A.M. – ORACLE Racing team press conference with CEO Russell Coutts and fellow Cup winners James Spithill and John Kostecki, with America’s Cup Principal Race Officer John Craig ● 1:00 P.M. – Media boat to take members of the press out on the water to see the AC45 wing-sailed catamaran in action on San Francisco Bay. This is the only time in 2011 that these next-generation America’s Cup boats will sail in San Francisco. -
Emirates Team New Zealand Setzt Beim America's Cup Auf PC-Based
| worldwide | new zealand PC-Control 01 | 2019 Das Emirates Team New Zealand konnte den America’s Cup bereits zum dritten Mal gewinnen. Beim 35. America’s Cup wurde mit Katamaranen des Typs AC50 (15 m Bootslänge) gesegelt. | PC-Control 01 | 2019 worldwide | new zealand Industrielle Steuerungstechnik bewährt sich auch im rauen Einsatz bei einer Segelregatta Emirates Team New Zealand setzt beim America’s Cup auf PC-based Control und EtherCAT Im Juni 2017 siegte das Emirates Team New Zealand beim 35. America’s Cup in Bermuda überzeugend mit 7:1 über das Oracle Team USA. Das Segelteam gewann im Rahmen der Qualifikationsveranstaltung auch die Louis Vuitton Trophy und schlug die Teams aus Großbritannien, Frankreich, Japan und Schweden. Als unerlässlicher Helfer für einen schnellen und präzisen Trimm – die Anpassung der Auftriebs-Foils sowie der Segelstellung bzw. des Segelprofils an Wind, Kurs und Seegang – war die PC- und EtherCAT-basierte Steuerungstechnik mit an Bord der Neuseeländer. Beckhoff ist nun offizieller Lieferant des Teams für die Cup- Verteidigung und kann daher über die Technik dieser ältesten noch heute ausgetragenen Segelregatta berichten. | worldwide | new zealand PC-Control 01 | 2019 Mit PC-based Control von Beckhoff lässt sich jede Funktion des Segelboots auch per Die schnelle, genaue Identifikation und Diagnose von Problemen sind entscheident Tablet über eine Webseite steuern. für eine Maximierung der Trainings- und Testzeiten auf dem Wasser. Beim Emirates Team New Zealand gibt es einige Anforderungen, die bei traditio- zwischen TwinCAT-ADS-Bibliotheken und Echtzeit-Steuerung – lokal und per nellen Industrieanwendungen in dieser Form nicht erforderlich sind. Notwendig Netzwerk – bedeutete für uns eine ultimative Flexibilität beim Management der sind kompakte, leichte Hochleistungssteuerungen, die hohen Temperaturen, Systemarchitektur. -
Seahorse International Sailing Guide to the America's
ContentsThereThere | Zoom in | Zoom out For navigation instructions please click here Search Issue | Next Page isis no no SecondSecond The Seahorse InternationalInternational SailingSailing guide to the America’s Cup PAUL CAYARD DENNIS CONNER RUSSELL COUTTS PAUL BIEKER MIRKO GROESCHNER TOM SCHNACKENBERG… AND FRIENDS in association with Contents | Zoom in | Zoom out For navigation instructions please click here Search Issue | Next Page A Seahorse Previous Page | Contents | Zoom in | Zoom out | Front Cover | Search Issue | Next Page EF MaGS International Sailing B You & Us Available in two locations. Everywhere, and right next to you. Because financial solutions have no borders or boundaries, UBS puts investment analysts in markets across the globe. We have specialists worldwide in wealth management, asset management and investment banking. So your UBS financial advisor can draw on a network of resources to provide you with an appropriate solution – and shrink the world to a manageable size. While the confidence you bring to your financial decisions continues to grow. You & Us. www.ubs.com___________ © UBS 2007. All rights reserved. A Seahorse Previous Page | Contents | Zoom in | Zoom out | Front Cover | Search Issue | Next Page EF MaGS International Sailing B A Seahorse Previous Page | Contents | Zoom in | Zoom out | Front Cover | Search Issue | Next Page EF MaGS International Sailing B WELCOME 3 Dear friends and fellow final of the America’s Cup. America’s Cup enthusiasts UBS is committed to the unique and dynamic sport of sailing as we This summer the America’s Cup, one represent the same values and skills of sport’s oldest and most prestigious required to succeed in global financial trophies, returns to Europe for the services: professionalism, teamwork, first time in over 150 years. -
Emirates Team New Zealand and the America's
Emirates Team New Zealand and the America’s Cup A real life context for STEM and innovative technology Genesis is proud to be supporting Emirates Team New Zealand as they work towards defending the 36th America’s Cup, right here in Auckland, New Zealand. Both organisations are known for their creative innovation and design solutions, so together they’ve developed sailing themed resources for the School-gen programme to inspire the next generation of Kiwi scientists, sailors, innovators and engineers. Overview These PDF posters, student worksheets, Quizzz quiz and teacher notes provide an introduction to: • A history of the America’s Cup • The history of sailing • How Genesis is powering Emirates Team New Zealand The resources described in the teacher notes are designed to complement the America’s Cup Trophy experience brought to schools by Genesis. Curriculum links: LEARNING AREAS LEVELS YEARS Science: Above: Physical World Emirates Team Physical inquiry and physics 3-4 5-8 New Zealand visit concepts Arahoe School Nature of Science: Communicating in Science Technology: Nature of Technology Characteristics of Technology 3-4 5-8 Technological Knowledge: Technological products @ Genesis School-gen Teacher information Learning sequence Introducing Explore and Create and Reflect and Make a difference knowledge investigate share extend Learning intentions Students are learning to: • explain how sailing technology has evolved over time • investigate solar power technology and energy • explore the history of the America’s Cup races and the America’s -
(Contents January 1999 FEATURES ^F'^Osl REGULARS
(Contents January 1999 FEATURES Farr 40 OD world championship and 1D35 US 31 Towards clarity and simplicity nationals reports, Route du Rhum fleet storm For some time it has been well known that there south, man-overboard row in Sydney, Macquarie were anomalies in the 1996-2000 rules, espe Innovation winding up to 50, Sydney-Hobart cially as applied to match racing. Team preview, Syd Fischer scores New Zealand have been pushing for America's Cup points (ashore), Coutts changes for some time, and recently returns to match-race circuit to take some were made. But is it time for a Bermuda Gold Cup, Cayard hits whole new approach? RUSSELL back at Bitter End Pro-Am, and the COUnS and TIM JEFFERY Mari-Cha transatlantic story - and , lessons for The Race 2000. With FRONT COVER: 34 mOB Itl lieaven? V Mm PATRICE CARPENTIER, IV^OR Mike Golding storms into It sorted its differences with ISAF, but \ WILKINS, ROB Cape Town on Team Group the ORC failed to address IMS stability at MUNDLE, DOBBS DAVIS, JOHN 4 to win leg one of the its annual round of meetings. ORC and ITC ROBERSON and ALASTAIR ABREHART Around Alone Race member DAVID LYONS reports from Palma and This opening performance p^UL HENDERSON gives his views on the ^ P||ii| RmmPÉ X%?"^rking En ^f'^oSL at the JMV shipyard in S^n 6o\^a?b"üt'Th'Zgl , '^c M^" '""^^'i TTM 24 Olympic AwA sDiall boat news Goldingl projec mlnag^ ÏJÏïpP v'^'"^ T T7 A ^on't take that old 49er to Sydney, nor your ment te'am'ieant hard on ^FFERY reports on eg one of he Around Europe wing-mast. -
At the Helm, I Am Confident This Will Continue in 2013
The Bahia Corinthian Yacht Club M Issue 10/10 - Volume 83 astheadDecember 2012 Commodore Winnett karen.winnett @klgates.com t th e elm I want to take this opportunity to express my H appreciation to the 2012 Executive Committee and their wonderfully supportive spouses.A It has been such a pleasure to be with a group who set their egos aside and worked so selflessly and collaboratively to support each other and achieve common goals. With Tom Madden at the helm, I am confident this will continue in 2013. My thanks to the 2012 board members for their patience in allowing me to make changes in our meeting processes so that they more closely mirror the running of a corporate board, improve the presentation of information needed for decisions and shorten the meetings. It is a pleasure to work with such a diverse group of people who are able to quickly reach consensus. I owe a debt of gratitude to the 2012 committee chairs and all of our volunteers. Without them, the club would not be what it is. They are often behind the scenes and their efforts should be recognized for their importance and appreciated by all of us who enjoy the club. We are fortunate that Scott Jones has joined us as general manager. The new employees whom Scott has hired are clear evidence that he is building a great new team to work alongside our longtime BCYC employees. It is with great pleasure that I welcome Tom Madden as incoming commodore. He will be fabulous and is sure to leave his mark on the club. -
2019 Section 9
West Virginia Annual Conference Journal 2019 Section IX - Roll of the Honored Dead IX. ROLL OF DEAD Clergy Members of the Conference (For deaths prior to 1969, see the 1969 Journal) Thomas E. Brooks ............................June 28, 1969 Ottie Clarence Mitchell ...................February 10, 1972 George W. Simpson..........................June 29, 1969 William Daniel Winters...................February 25, 1972 P. L. O’Dell ......................................... July 5,1969 Woodrow Lee Haught (AM) .................April 18, 1972 W. W. Bragg ................................ August 22, 1969 Frederick Oxendale ..................................May 3, 1972 Frank E. Perry ..........................September 2, 1969 Rowland Aspinall ...................................May 16, 1972 Glenn D. Watts .......................September 17, 1969 Edward J. Heller .....................................June 21, 1972 J. Herbert Parks ..........................October 19, 1969 Thomas E. Shea ..................................... July 15, 1972 W. W. Beckley ...........................December 4, 1969 Walter Overstreet ................................... July 27, 1972 John Baptist Staley ....................December 8, 1969 Joseph T. Tisdale (AM) ....................... August 3, 1972 Arthur B. Moore ......................December 21, 1969 Charles W. Pugh .................................August 11, 1972 Arthur S. Carter .............................January 1, 1970 Daniel L. Snyder ..........................September 14, 1972 Randall Hamrick ...........................January -
Governing Body Meeting Held on 23/11/2017
America’s Cup 36 Location Analysis – Full Technical Report Version 1.1 16 November 2017 America’s Cup 36 Location and Infrastructure work stream Document history Version Date Author Update details 1.1 15/11/17 Fiona Knox, Strategic Project Manager. Panuku Final Document review Role Name and signature Date Panuku Director Design + Place Rod Marler Panuku Chief Operating Officer David Rankin Auckland Council CEO Stephen Town ii America’s Cup 36 Location and Infrastructure work stream Table of Contents Introduction ................................................................................................................... 1 Vision for 2021 .................................................................................................................... 1 Location analysis work stream ............................................................................................ 1 Purpose of this document ................................................................................................... 3 Report structure .................................................................................................................. 3 Process .......................................................................................................................... 4 ILM workshop ..................................................................................................................... 4 Assessment criteria - identification ...................................................................................... 4 Assessment -
Contenuti 1. Prefazione Di Sir Peter Blake 2. America's Cup History 3
Contenuti 1. Prefazione di Sir Peter Blake 2. America's Cup History 3. Luna Rossa History 4. Il team: 4.1 Patrizio Bertelli - Team Principal 4.2 I componenti del team 5. La sfida alla 35^ America's Cup 6. Le America’s Cup World Series 7. Le barche: 7.1 I catamarani classe AC45 7.2 I catamarani classe AC62 8. La base di Cagliari 9. Il Circolo della Vela Sicilia 10. Sponsor 10.1 Prada 11. Fornitori Ufficiali: 11.1 ABC Tools 11.2 CR24 11.3 ESTECO 11.4 Lenovo 11.5 Sanpellegrino 11.6 Si14 11.7 Technogym Marzo 2015 1. Prefazione di Sir Peter Blake al libro "Luna Rossa" sulla 30^ America's Cup (2000) La Coppa America è un trofeo molto ambito, ma che di rado ha cambiato mano in 150 anni. Questo non è uno sport per deboli di cuore. Non è impresa da prendere alla leggera o per capriccio. É una lotta tra velisti di Yacht Club sparsi nel mondo che vogliono disperatamente la stessa cosa: mettere le mani sulla Coppa. Il prestigio per il vincitore vale più di qualsiasi altro riconoscimento sportivo. É proprio vincere l’invincibile e fare l’impossibile che affascina uomini di mare, sognatori e miliardari. Ma la vittoria non arriva facilmente. Anzi, il più delle volte non arriva affatto. L’unico modo per vincere è continuare a partecipare, continuare a tornare, una volta dopo l’altra, con l’intimo convincimento di potercela fare. Esitare dopo il primo tentativo non fa parte delle regole del gioco. Ci vogliono persone straordinarie, con una motivazione ferrea, grande esperienza, attenzione per i particolari e dedizione incondizionata. -
Heart of Ice from the Green Fairy Book by Andrew Lang, Ed. Once
Heart of Ice 'Oh! prate away,' said she, 'your son will never be from The Green Fairy Book anything to boast of. Say what you will, he will be by Andrew Lang, Ed. nothing but a Mannikin--' No doubt she would have gone on longer in this Once upon a time there lived a King and Queen who strain, and given the unhappy little Prince half-a- were foolish beyond all telling, but nevertheless they dozen undesirable gifts, if it had not been for the were vastly fond of one another. It is true that certain good Fairy Genesta, who held the kingdom under her spiteful people were heard to say that this was only special protection, and who luckily hurried in just in one proof the more of their exceeding foolishness, time to prevent further mischief. When she had by but of course you will understand that these were not compliments and entreaties pacified the unknown their own courtiers, since, after all, they were a King Fairy, and persuaded her to say no more, she gave the and Queen, and up to this time all things had King a hint that now was the time to distribute the prospered with them. For in those days the one thing presents, after which ceremony they all took their to be thought of in governing a kingdom was to keep departure, excepting the Fairy Genesta, who then well with all the Fairies and Enchanters, and on no went to see the Queen, and said to her: account to stint them of the cakes, the ells of ribbon, and similar trifles which were their due, and, above 'A nice mass you seem to have made of this business, all things, when there was a christening, to remember madam. -
Memoirs of Hydrography
MEMOIRS 07 HYDROGRAPHY INCLUDING Brief Biographies of the Principal Officers who have Served in H.M. NAVAL SURVEYING SERVICE BETWEEN THE YEARS 1750 and 1885 COMPILED BY COMMANDER L. S. DAWSON, R.N. I 1s t tw o PARTS. P a r t II.—1830 t o 1885. EASTBOURNE: HENRY W. KEAY, THE “ IMPERIAL LIBRARY.” iI i / PREF A CE. N the compilation of Part II. of the Memoirs of Hydrography, the endeavour has been to give the services of the many excellent surveying I officers of the late Indian Navy, equal prominence with those of the Royal Navy. Except in the geographical abridgment, under the heading of “ Progress of Martne Surveys” attached to the Memoirs of the various Hydrographers, the personal services of officers still on the Active List, and employed in the surveying service of the Royal Navy, have not been alluded to ; thereby the lines of official etiquette will not have been over-stepped. L. S. D. January , 1885. CONTENTS OF PART II ♦ CHAPTER I. Beaufort, Progress 1829 to 1854, Fitzroy, Belcher, Graves, Raper, Blackwood, Barrai, Arlett, Frazer, Owen Stanley, J. L. Stokes, Sulivan, Berard, Collinson, Lloyd, Otter, Kellett, La Place, Schubert, Haines,' Nolloth, Brock, Spratt, C. G. Robinson, Sheringham, Williams, Becher, Bate, Church, Powell, E. J. Bedford, Elwon, Ethersey, Carless, G. A. Bedford, James Wood, Wolfe, Balleny, Wilkes, W. Allen, Maury, Miles, Mooney, R. B. Beechey, P. Shortland, Yule, Lord, Burdwood, Dayman, Drury, Barrow, Christopher, John Wood, Harding, Kortright, Johnson, Du Petit Thouars, Lawrance, Klint, W. Smyth, Dunsterville, Cox, F. W. L. Thomas, Biddlecombe, Gordon, Bird Allen, Curtis, Edye, F.