Revealing the Diverse Magnetic Field Morphologies in Taurus Dense Cores with Sensitive Sub-Millimeter Polarimetry
Total Page:16
File Type:pdf, Size:1020Kb
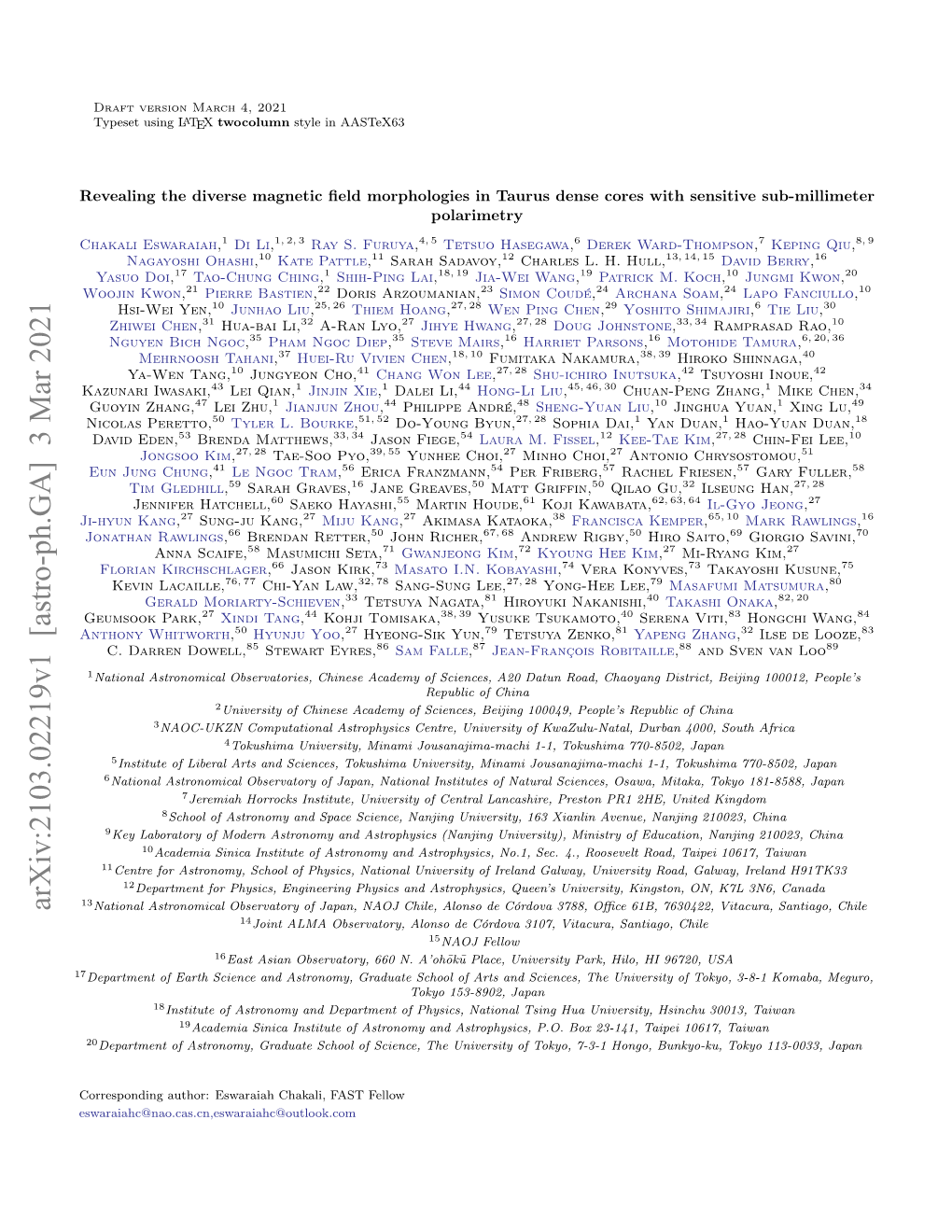
Load more
Recommended publications
-
Report on the Radionet3 Networking Activity
REPORT ON THE RADIONET3 NETWORKING ACTIVITY TITLE: LOFAR SCIENCE WEEK 2014 A JOINT MEETING COMBINING THE “2014 LOFAR COMMUNITY SCIENCE WORKSHOP” AND A SEPARATE, RELATED SYMPOSIUM ON “FIRST SCIENCE WITH LOFAR’S FIRST ALL-SKY SURVEY” DATE: 7 APRIL – 11 APRIL 2014 TIME: ALL DAY LOCATION: AMSTERDAM, THE NETHERLANDS MEETING WEBPAGE http://www.astron.nl/lofarscience2014/ HOST INSTITUTE: ASTRON (NETHERLANDS INSTITUTE FOR RADIO ASTRONOMY) PARTICIPANTS NO: 105 (COMMUNITY WORKSHOP) / 62 (SURVEY WORKSHOP) MAIN LEADER: ASTRON Project supported by the European Commission Contract no.: 283393 REPORT: 1. Agenda of the meeting The final programmes for both of the workshops are reproduced in their entirety in Appendix A. The programs and presentations are also available from the conference website. 2. Scientific Summary The LOFAR Science Week for 2014 was held April 7-11, 2014 in Amsterdam, NL and brought together roughly 120 members of the LOFAR science community. The week began on Monday afternoon with a LOFAR Users Meeting, open to the whole LOFAR community, organized by ASTRON and intended to provide a forum for users to both learn about the status of the array as well as provide feedback. Members of ASTRON gave updates on the current operational status, ongoing developments, and plans for the coming year. Representative users from the community were also invited to share their personal experiences from using the system. Robert Pizzo and the Science Support team were on hand to answer questions and gathered a lot of good feedback that they will use to improve the user experience for LOFAR. The User’s Meeting was followed on Tuesday by a two day LOFAR Community Science Workshop where nearly 120 members of the LOFAR collaboration came together to present their latest science results and share ideas and experiences about doing science with LOFAR. -
Planck 2011 Conference the Millimeter And
PLANCK 2011 CONFERENCE THE MILLIMETER AND SUBMILLIMETER SKY IN THE PLANCK MISSION ERA 10-14 January 2011 Paris, Cité des Sciences Monday, January 10th 2011 13:00 Registration 14:00 Claudie Haigneré (Présidente of Universcience) Welcome Planck mission status, Performances, Cross-calibration Jan Tauber (ESA) - Jean-Loup Puget (IAS Orsay) - Reno Mandolesi (INAF/IASF Bologna) Introduction Session chair: François Pajot 14:20 François Bouchet (Institut d'Astrophysique de Paris) 30+5' HFI data and performance (invited on Planck early paper) 14:55 Marco Bersanelli (University of Milano) 30+5' LFI data and performance (invited on Planck early paper) 15:30 Ranga Chary (IPAC Caltech) 30+5' Planck Early Release Compact Source Catalogue (invited on Planck early paper) 16:05 Coffee break 16:30 Goran Pilbratt (European Space Agency) 20+5' The Herschel observatory (invited) Radio sources 16:55 Bruce Partridge (Haverford College) 25+5' Overview (invited) 17:25 Luigi Tofolatti (Universidad de Oviedo) 20+5' Planck radio sources statistics (invited on Planck early paper) 17:50 Francisco Argueso (Universidad de Oviedo) 15+5' A Bayesian technique to detect point sources in CMB maps 18:10 Anna Scaife (Dublin Institute for Advanced Studies) 15+5' The 10C survey of Radio Sources - First Results 18:30 End of day 1 19:00 Welcome reception at the Conference Center Tuesday, January 11th 2011 Radio sources (continued) Session chair: Bruce Partridge 9:00 Anne Lahteenmaki (Aalto University Metsahovi Radio Observatory) 20+5' Planck observations of extragalactic radio -
Probing Galaxy Halos Using Background Polarized Radio Sources
Probing galaxy halos using background polarized radio sources Master’s thesis in Physics and Astronomy ANTON NILSSON Department of Earth and Space Sciences CHALMERS UNIVERSITY OF TECHNOLOGY Gothenburg, Sweden 2016 Probing galaxy halos using background polarized radio sources Anton Nilsson Department of Earth and Space Sciences Radio Astronomy and Astrophysics group Chalmers University of Technology Gothenburg, Sweden 2016 Probing galaxy halos using background polarized radio sources Anton Nilsson © Anton Nilsson, 2016. Supervisor: Cathy Horellou, Department of Earth and Space Sciences Examiner: Cathy Horellou, Department of Earth and Space Sciences Department of Earth and Space Sciences Radio Astronomy and Astrophysics group Chalmers University of Technology SE-412 96 Gothenburg Telephone +46 31 772 1000 Cover: Polarized emission of the radio source J133920+464115 at Faraday depth +20.75 rad m−2 in grayscale. The contours show the total intensity. Typeset in LATEX Printed by Chalmers Reproservice Gothenburg, Sweden 2016 iv Probing galaxy halos using background polarized radio sources Anton Nilsson Department of Earth and Space Sciences Chalmers University of Technology Abstract Linearly polarized radio emission from synchrotron sources undergoes Faraday ro- tation when passing through a magneto-ionized medium. This might be used to search for evidence of ionized halos around galaxies, using polarized radio sources as a probe. In this thesis I analyze the polarization properties of background radio sources from the Taylor et al. (2009) catalog based on observations with the Very Large Array, and correlate these to the angular separation between the radio sources and foreground galaxies. I find a decrease in the amount of polarized radio sources near the galaxies, interpreting this as radio sources being depolarized by galaxy halos. -
OTFM for Fast Mapping & Rapid Data Products
OTFM for fast mapping & rapid data products Steven T. Myers (NRAO) for the VLASS team 1 B&E Caltech July 2016 The VLA Sky Survey (VLASS) On-the-fly Mosaicking (OTFM) for efficient fast mapping of the sky and pipelines for rapid genera=on of Data Products … wherein we elucidate the sundry benefits of using the recently upgraded Karl G. Jansky Very Large Array to survey large areas of the sky to discover new transient phenomena and illuminate heretofore hidden explosions throughout the Universe, and to explore cosmic magnetism h0ps://science.nrao.edu/science/surveys/vlass 2 B&E Caltech July 2016 Why a new VLA Sky Survey? A New VLA deserves a New Sky Survey https://science.nrao.edu/science/surveys/vlass • Expanded (bandwidth, time resolution) capabilities of Jansky VLA • 20 years since previous pioneering VLA sky surveys – NVSS 45” 1.4GHz (84MHz) 450µJy/bm (2932hrs) 30Kdeg2 2Msrcs – FIRST 5” 1.4GHz (84MHz) 150µJy/bm (3200hrs) 10.6Kdeg2 95Ksrcs • Preparing the way for a new generation of surveys – ASKAP and MeerKAT – SKA phase 1 – new O/IR surveys (i/zPTF, PanSTARRS,DES,LSST), X-ray (eROSITA) • Science Proposal and survey definition by community Survey Science Group (SSG) following AAS 223 workshop in Jan 2014 – community review and approval in March 2015 3 B&E Caltech July 2016 VLASS Survey Science Group A Survey For the Whole Community Proposal Authors: Table 8: VLASS Proposal Contributors, Including VLASS White Paper Authors F. Abdalla, Jose Afonso, A. Amara, David Bacon, Julie Banfield, Tim Bastian, Richard Battye, Stefi A. Baum, Tony Beasley, Rainer Beck, Robert Becker, Michael Bell, Edo Berger, Rob Beswick, Sanjay Bhat- nagar, Mark Birkinshaw, V. -
Square Kilometre Array: Processing Voluminous Meerkat Data on IRIS
SQUARE KILOMETRE ARRAY :PROCESSING VOLUMINOUS MEERKAT DATA ON IRIS Priyaa Thavasimani Anna Scaife Faculty of Science and Engineering Faculty of Science and Engineering The University of Manchester The University of Manchester Manchester, UK Manchester, UK [email protected] [email protected] June 1, 2021 ABSTRACT Processing astronomical data often comes with huge challenges with regards to data management as well as data processing. MeerKAT telescope is one of the precursor telescopes of the World’s largest observatory Square Kilometre Array. So far, MeerKAT data was processed using the South African computing facility i.e. IDIA, and exploited to make ground-breaking discoveries. However, to process MeerKAT data on UK’s IRIS computing facility requires new implementation of the MeerKAT pipeline. This paper focuses on how to transfer MeerKAT data from the South African site to UK’s IRIS systems for processing. We discuss about our RapifXfer Data transfer framework for transferring the MeerKAT data from South Africa to the UK, and the MeerKAT job processing framework pertaining to the UK’s IRIS resources. Keywords Big Data · MeerKAT · Square Kilometre Array · Telescope · Data Analysis · Imaging Introduction The Square Kilometre Array (SKA) will be the world’s largest telescope, but once built it comes with its huge data challenges. The SKA instrument sites are in Australia and South Africa. This paper focuses on the South African SKA precursor MeerKAT, in particular on its data processing, calibration, and imaging using the UK IRIS resources. MeerKAT data are initially hosted by IDIA (Inter-University Institute for Data-Intensive Astronomy; [1]), which itself provides data storage and a data-intensive research cloud facility to service the MeerKAT science community in South Africa. -
Data Science for Physics and Astronomy Lightning Talks Data Science for Physics & Astronomy Classification of Transients Catarina Alves
Data science for Physics and Astronomy Lightning talks Data science for Physics & Astronomy Classification of transients Catarina Alves PhD @ Supervisors: ● Prof Hiranya Peiris ● Dr Jason McEwen [email protected] Supervised Learning Classification Unbalanced data Non representative data catarina-alves Catarina-Alves Unsupervised Learning Real time classification Anomaly detection Large datasets 2 Data science for Physics & Astronomy Adrian Bevan Particle physics experimentalist with a keen interest in DS & AI Parameter estimation: ● 2 decades of likelihood fitting experience spanning NA48, BaBar and ATLAS experiments; currently using ML on the ATLAS and MoEDAL experiments @ LHC. ● Latest relevant work: https://royalsocietypublishing.org/doi/10.1098/rsta.2019.0392 Machine learning: ● Broad interest in algorithms: domains of validity to understand what to use when and why to optimise the use of algorithms for a given problem domain. Have used SVMs, BDTs, MLPs, CNNs, Deep Networks, KNN for analysis work. ● Working on understanding why algorithms make a given inference or decision based on input feature space (explainability and interpretability). ● Want to explore unsupervised learning and bayesian networks when an appropriate problem presents itself. Have taught or currently teach data science and machine learning undergrad and grad courses. [email protected] 3 Data science for Physics & Astronomy David Berman 4 Data science for Physics & Astronomy Richard Bielby 5 Data science for Nachiketa Chakraborty Physics & Astronomy -
The Very Small Array: Latest Results and Future Plans
The Very Small Array: latest results and future plans Keith Grainge Astrophysics Group, Cavendish Laboratory, Cambridge Rencontres de Moriond, 31 March 2004 OVERVIEW The Very Small Array (VSA) • Current primordial observations • The S-Z effect • Planned upgrades to the instrument • Future science at high ` • Summary • 1 THE VSA CONSORTIUM Cavendish Astrophysics Group Roger Boysen Tony Brown Chris Clementson Mike Crofts Jerry Czeres Roger Dace Keith Grainge (PM) Mike Hobson (PI) Mike Jones Rüdiger Kneissl Katy Lancaster Anthony Lasenby Klaus Maisinger Ian Northrop Guy Pooley Vic Quy Nutan Rajguru Ben Rusholme Richard Saunders Richard Savage Anna Scaife Jack Schofield Paul Scott (Former PI) Clive Shaw Anze Slosar Angela Taylor David Titterington Elizabeth Waldram Brian Wood Jodrell Bank Observatory Colin Baines Richard Battye Eddie Blackhurst Pedro Carreira Kieran Cleary Rod Davies Richard Davis Clive Dickinson Yaser Hafez Mark Polkey Bob Watson Althea Wilkinson Instituto de Astrofísica de Canarias Jose Alberto Rubiño-Martin Carlos Guiterez Rafa Rebolo Lopez Pedro Sosa-Molina Ricardo Genova-Santos 2 THE VERY SMALL ARRAY (VSA) Sited on Observatorio del Teide, Tenerife • 14 antennas 91 baselines • ) Observing frequency ν = 26 36 GHz; bandwidth ∆ν = 1:5 GHz • − Compact configuration: 143 mm horns; ` = 150 800; ∆` 90 (without • − ≈ mosaicing); Sept 2000 – Sept 2001 Extended configuration: 322 mm horns; ` = 300 1800; ∆` 200 (without • − ≈ mosaicing); Sept 2001 – present 3 COMPARISON WITH OTHER CMB EXPERIMENTS Different systematics from “single-dish” experiments: • – negligible pointing error – negligible beam uncertainty Different from other CMB interferometers (DASI, CBI): • – RT survey and dedicated source subtraction 2-element interferometer remove effect of extragalactic point sources ) – tracking elements rather than comounted can apply fringe rate filter to remove unwanted signals. -
Researcher Exchanges in Radio Astronomy – University of Manchester Projects January 2019
Researcher Exchanges in Radio Astronomy – University of Manchester projects January 2019 1. Unveiling star formation across cosmic time: Predictions for the SKA Supervisor: Dr Rowan Smith The SKA will revolutionise our understanding of how galaxies form and transform their gas into stars by mapping HI 21cm line emission with exquisite detail and sensitivity. With the SKA it will be possible to peer back across cosmic time and study the interstellar medium (ISM) in a whole range of galaxy types and metallicities, including irregular dwarf galaxies such as those found in the early universe. However, to truly unlock the power of such observations it is crucial to have similarly advanced models to compare the data too. In this project we will use cutting edge simulations of the ISM in different galaxy types with the Arepo MHD code to study where atomic hydrogen gas is located and when it transforms into molecular H2. The simulations are resolved down to sub-pc scales and include a detailed model of the chemistry of the warm and cold gas. Using these simulations we will perform post-process radiative transfer simulations with the Polaris and LIME codes to predict the HI emission that would be seen from different galaxy types with the SKA. In order to get a full view of the gas life-cycle in such galaxies we will then also calculate the molecular gas emission that would be seen in CO with ALMA, and the CII emission that could be seen in nearby galaxies with SOFIA. Using our simulations and these synthetic emission maps we will aim to make testable predictions about the evolution of galaxies from low-metallicity dwarfs, to grand design spirals, to star-burst galaxies and how they transform their gas into stars. -
Advancing Astrophysics with the Square Kilometre Array
Advancing Astrophysics with the Square Kilometre Array 8-13 June 2014 Giardini Naxos, Italy #skascicon14 Abstracts Book ISTITUTO NAZIONALE DI ASTROFISICA INAF NATIONAL INSTITUTE FOR ASTROPHYSICS SESSION 1: Chair – Jonathan Pritchard The Cosmic Dawn and Epoch of Reionisation with SKA Leon Koopmans1;*, and the EoR Working Group 1 Kapteyn Astronomical Institute, University of Groningen, The Netherlands * Presenter E-mail contact: koopmans at astro.rug.nl Concerted effort is currently ongoing to open up the Epoch of Reionization (z 15- Ø 6) for studies with IR and radio telescopes. Whereas IR detections have been made of sources (Ly- emitters, quasars and drop-outs) in this redshift regime, in relatively small fields of view, no direct detection of neutral hydrogen via the redshifted 21-cm line has yet been established. Such a direct detection is expected in the coming years with ongoing surveys and will open up the entire universe from z 6-200 for astrophysical Ø and cosmological studies, opening not only the EoR, but also its preceding Cosmic Dawn (z 40-15) and possibly even the later phases of the Dark Ages (z 200-40), leaving Ø Ø only the “Age of Ignorance” (z 1100-200) inaccessible to astronomers. All currently Ø ongoing experiments attempt statistical detections of the 21-cm signal during the EoR, with limited signal-tonoise. Direct imaging, except maybe on the largest (degree) scales at lower redshift, will remain out of reach. The Square Kilometre Array (SKA), however, will revolutionize the field, and allow direct imaging of neutral hydrogen from scales of arc-minutes to degrees over most of the redshift range z 6-30. -
Delivering a Mega-Science Project for the World
THE SKA OBSERVATORY DELIVERING A MEGA-SCIENCE PROJECT FOR THE WORLD SKAO PROSPECTUS 2020 “With the SKA, you see how Contents astronomy brings together the Introduction: Next-generation radio astronomy 5 worlds of science and diplomacy. 1 SKAO - the SKA Observatory 13 2 Science 17 It shows that you can have inclusive 3 Impact 21 scientific, cultural and societal 4 Big Data 30 5 Establishment and delivery of the Observatory 32 development all together.” 6 Construction 34 7 Baseline budget 38 8 Baseline schedule 39 Dr Marga Gual Soler Science Diplomacy Expert and 2020 Young Global Leader of the World Economic Forum 2 3 SKAO PROSPECTUS 2020 SKAO PROSPECTUS 2020 Next-generation radio astronomy The Square Kilometre Array (SKA) is a One array of 197 dishes, each 15m next-generation radio astronomy-driven in diameter, with a 150km maximum Big Data facility that will revolutionise our separation between the most distant understanding of the Universe and the dishes, located in South Africa. SKAO Global Headquarters laws of fundamental physics. Enabled by One array of 131,072 smaller antennas in the UK is home to cutting-edge technology, it promises to more than 120 experts grouped in 512 stations, with up to have a major impact on society, in science in science, engineering, 65km maximum separation between project management, policy, and beyond. the most distant stations, located in international law and many This publication presents a €1.99 Western Australia. other specialisms. The purpose-built HQ is neighbour billion (2020 €), 10-year project for the to Jodrell Bank Observatory, a construction and early operations of UNESCO World Heritage Site. -
Convening Innovators from the Science and Technology Communities Annual Meeting of the New Champions 2014
Global Agenda Convening Innovators from the Science and Technology Communities Annual Meeting of the New Champions 2014 Tianjin, People’s Republic of China 10-12 September Discoveries in science and technology are arguably the greatest agent of change in the modern world. While never without risk, these technological innovations can lead to solutions for pressing global challenges such as providing safe drinking water and preventing antimicrobial resistance. But lack of investment, outmoded regulations and public misperceptions prevent many promising technologies from achieving their potential. In this regard, we have convened leaders from across the science and technology communities for the Forum’s Annual Meeting of the New Champions – the foremost global gathering on innovation, entrepreneurship and technology. More than 1,600 leaders from business, government and research from over 90 countries will participate in 100- plus interactive sessions to: – Contribute breakthrough scientific ideas and innovations transforming economies and societies worldwide – Catalyse strategic and operational agility within organizations with respect to technological disruption – Connect with the next generation of research pioneers and business W. Lee Howell Managing Director innovators reshaping global, industry and regional agendas Member of the Managing Board World Economic Forum By convening leaders from science and technology under the auspices of the Forum, we aim to: – Raise awareness of the promise of scientific research and highlight the increasing importance of R&D efforts – Inform government and industry leaders about what must be done to overcome regulatory and institutional roadblocks to innovation – Identify and advocate new models of collaboration and partnership that will enable new technologies to address our most pressing challenges We hope this document will help you contribute to these efforts by introducing to you the experts and innovators assembled in Tianjin and their fields of research. -
Rapidxfer: Proposed Data Transfer Framework for Square Kilometre Array
RapidXfer: Proposed Data Transfer Framework for Square Kilometre Array - Dr Priyaa Thavasimani, Prof Anna Scaife, Jodrell Bank Centre for Astrophysics, The University of Manchester Introduction to SKA • World's largest radio telescope project – sites Australia (ASKAP) and South Africa (MeerKAT) - integrated into Phase 1 of SKA. • The volume of telescope data is vast. • data rate as approximately 23 Tb/s from the antennas to the correlator, 14 Tb/s from the correlator to the HPC (provided by SDP). • Expected volume of data to partner countries – 600 PB / year • 15 member countries. What is MeerKAT? • Karoo Array Telescope • radio telescope consisting of 64 antennas in the Northern Cape of South Africa, inaugurated in 13 July 2018. • a precursor for the SKA MeerKAT Data transfer from IDIA to IRIS • IDIA (Inter-University Institute for Data Intensive Astronomy) intends to provide researchers from South Africa with cloud resources to process Astronomical Big Data. • IRIS funded by the STFC (one of research council in UKRI) - create and develop the digital research infrastructure - computing related resources needed to support science. • Datasets (of 1.3 TB approx each) to be transferred from IDIA to IRIS. • Globus online – secure, reliable service, enables you to share and transfer files faster than traditional scp and rsync transfers. Containerised DiRAC Environment on IDIA • SKA UK is currently using WLCG DMS/WMS services in particular DiRAC. • Containerised DiRAC Environment on IDIA • dirac-dms-add-file functionality facilitates transfer of smaller files. RapidXfer - Data Transfer and Processing Framework Globus Transfer of 1.33 TB Data Data Transfer Speed from IDIA to IRIS (Approximate Calculation) • (Globus transfer) 1 TB = 18.79 hours Gfal-copy = 0.829 hours Total = 19.56 hours Total Data rate: 14.20 MB/sec Note: Represents average repeatable performance, i.e.