Hydrothermal Organic Reduction and Deoxygenation
Total Page:16
File Type:pdf, Size:1020Kb
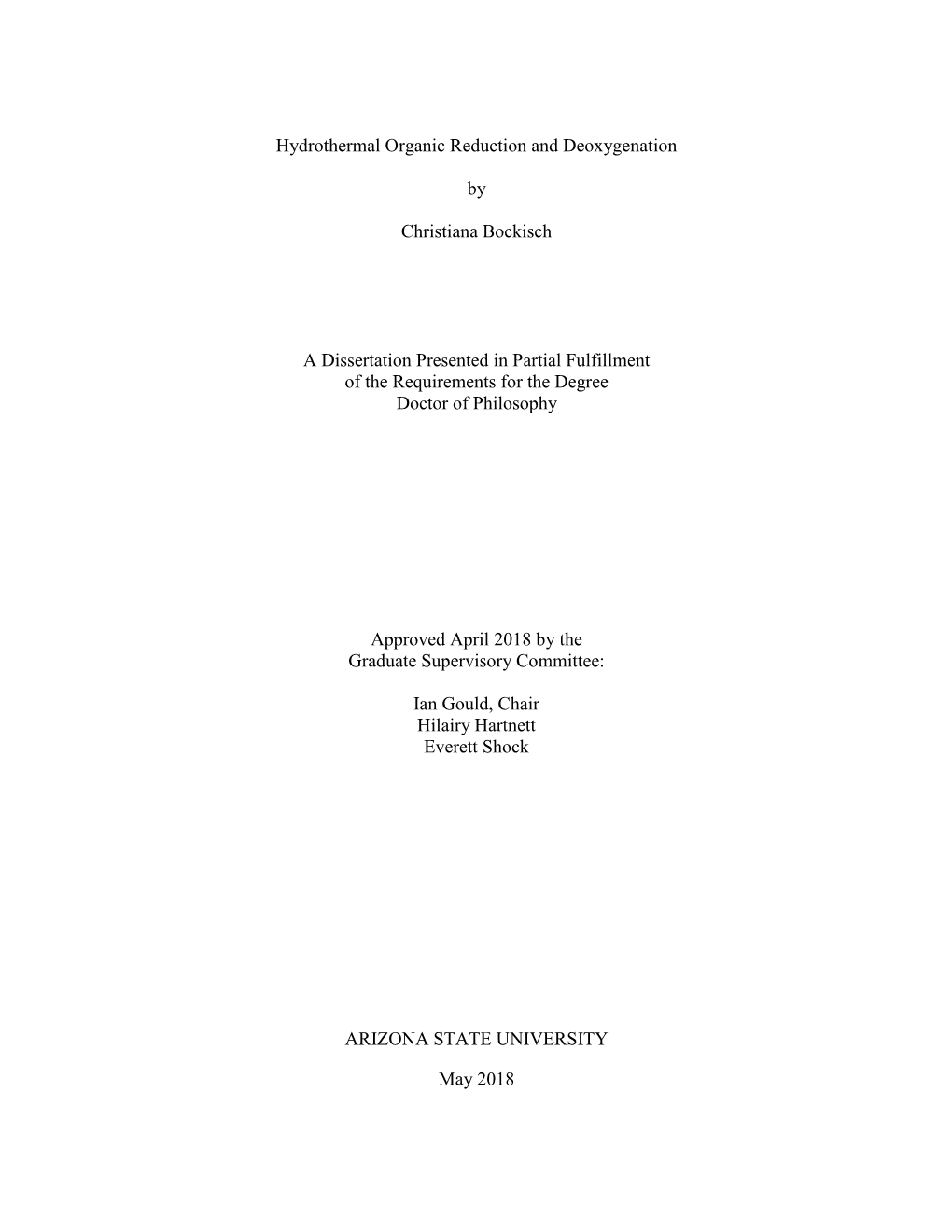
Load more
Recommended publications
-
Modern-Reduction-Methods.Pdf
Modern Reduction Methods Edited by Pher G. Andersson and Ian J. Munslow Related Titles Yamamoto, H., Ishihara, K. (eds.) Torii, S. Acid Catalysis in Modern Electroorganic Reduction Organic Synthesis Synthesis 2008 2006 ISBN: 978-3-527-31724-0 ISBN: 978-3-527-31539-0 Roberts, S. M. de Meijere, A., Diederich, F. (eds.) Catalysts for Fine Chemical Metal-Catalyzed Cross- Synthesis V 5 – Regio and Coupling Reactions Stereo-Controlled Oxidations 2004 and Reductions ISBN: 978-3-527-30518-6 2007 Online Book Wiley Interscience Bäckvall, J.-E. (ed.) ISBN: 978-0-470-09024-4 Modern Oxidation Methods 2004 de Vries, J. G., Elsevier, C. J. (eds.) ISBN: 978-3-527-30642-8 The Handbook of Homogeneous Hydrogenation 2007 ISBN: 978-3-527-31161-3 Modern Reduction Methods Edited by Pher G. Andersson and Ian J. Munslow The Editors All books published by Wiley-VCH are carefully produced. Nevertheless, authors, editors, and Prof. Dr. Pher G. Andersson publisher do not warrant the information Uppsala University contained in these books, including this book, to Department of Organic Chemistry be free of errors. Readers are advised to keep in Husargatan 3 mind that statements, data, illustrations, 751 23 Uppsala procedural details or other items may Sweden inadvertently be inaccurate. Dr. Ian J. Munslow Library of Congress Card No.: Uppsala University applied for Department of Biochemistry and Organic Chemistry Husargatan 3 British Library Cataloguing-in-Publication Data 751 23 Uppsala A catalogue record for this book is available from Sweden the British Library. Bibliographic information published by the Deutsche Nationalbibliothek Die Deutsche Nationalbibliothek lists this publication in the Deutsche Nationalbibliografi e; detailed bibliographic data are available on the Internet at <http://dnb.d-nb.de>. -
Precursors and Chemicals Frequently Used in the Illicit Manufacture of Narcotic Drugs and Psychotropic Substances 2017
INTERNATIONAL NARCOTICS CONTROL BOARD Precursors and chemicals frequently used in the illicit manufacture of narcotic drugs and psychotropic substances 2017 EMBARGO Observe release date: Not to be published or broadcast before Thursday, 1 March 2018, at 1100 hours (CET) UNITED NATIONS CAUTION Reports published by the International Narcotics Control Board in 2017 The Report of the International Narcotics Control Board for 2017 (E/INCB/2017/1) is supplemented by the following reports: Narcotic Drugs: Estimated World Requirements for 2018—Statistics for 2016 (E/INCB/2017/2) Psychotropic Substances: Statistics for 2016—Assessments of Annual Medical and Scientific Requirements for Substances in Schedules II, III and IV of the Convention on Psychotropic Substances of 1971 (E/INCB/2017/3) Precursors and Chemicals Frequently Used in the Illicit Manufacture of Narcotic Drugs and Psychotropic Substances: Report of the International Narcotics Control Board for 2017 on the Implementation of Article 12 of the United Nations Convention against Illicit Traffic in Narcotic Drugs and Psychotropic Substances of 1988 (E/INCB/2017/4) The updated lists of substances under international control, comprising narcotic drugs, psychotropic substances and substances frequently used in the illicit manufacture of narcotic drugs and psychotropic substances, are contained in the latest editions of the annexes to the statistical forms (“Yellow List”, “Green List” and “Red List”), which are also issued by the Board. Contacting the International Narcotics Control Board The secretariat of the Board may be reached at the following address: Vienna International Centre Room E-1339 P.O. Box 500 1400 Vienna Austria In addition, the following may be used to contact the secretariat: Telephone: (+43-1) 26060 Fax: (+43-1) 26060-5867 or 26060-5868 Email: [email protected] The text of the present report is also available on the website of the Board (www.incb.org). -
Lanthanide Replacement in Organic Synthesis: Calcium-Mediated Luche-Type Reduction of Α,Β-Functionalised Ketones
Lanthanide Replacement in Organic Synthesis: Calcium-mediated Luche-type Reduction of α,β-functionalised Ketones A thesis submitted by Nina Viktoria Forkel In partial fulfilment of the requirement for a degree of Doctor of Philosophy Imperial College London Department of Chemistry South Kensington Campus SW7 2AZ London United Kingdom September 2013 Lanthanide Replacement in Organic Synthesis: Calcium-mediated Luche-type Reduction of α,β-functionalised Ketones “Man kann sich die Weite und Möglichkeiten des Lebens gar nicht unerschöpflich genug denken.” (Rainer Maria Rilke) This thesis is dedicated to all my loved ones. Declaration of originality and statement of copyright Declaration of originality I, Nina V. Forkel, certify that the research described within this thesis was carried out in the Department of Chemistry at Imperial College London between October 2009 and September 2012. It was accomplished under the primary supervision of Dr Matthew J. Fuchter, Imperial College London, along with supervision from Dr David A. Henderson, Pfizer Ltd. The entire body of work is that of the author, unless otherwise stated to the contrary, and has not been submitted previously for a degree at this or any other university. Statement of copyright The copyright of this thesis rests with the author and is made available under a Creative Commons Attribution Non-Commercial No Derivatives licence. Researchers are free to copy, distribute or transmit the thesis on the condition that they attribute it, that they do not use it for commercial purposes, and that they do not alter, transform or build upon it. For any reuse or redistribution, researchers must make clear to others the licence terms of this work. -
Safrole and the Versatility of a Natural Biophore Lima, L
Artigo Safrole and the Versatility of a Natural Biophore Lima, L. M.* Rev. Virtual Quim., 2015, 7 (2), 495-538. Data de publicação na Web: 31 de dezembro de 2014 http://www.uff.br/rvq Safrol e a Versatilidade de um Biofóro Natural Resumo: Safrol (1) obtido de óleos essenciais de diferentes espécies vegetais tem ampla aplicação na indústria química como precursor sintético do butóxido de piperonila, piperonal e de fármacos como a tadalafila, cinoxacina e levodopa. Do ponto vista toxicológico, é considerado uma hepatotoxina por mecanismo de bioativação metabólica conduzindo a formação de intermediários eletrofílicos, e tem sido descrito como inibidor de diferentes isoenzimas da família CYP450. A versatilidade de sua estrutura, permitindo várias transformações químicas, e a natureza biofórica de sua unidade benzodioxola ou metilenodioxifenila conferem-lhe características singulares, que o tornam atrativo material de partida para a síntese de compostos com distintas atividades farmacológicas. Exemplos selecionados de compostos bioativos, naturais e sintéticos, contendo o sistema benzodioxola serão comentados, incluindo aqueles provenientes de contribuição específica do LASSBio®. Palavras-chave: Safrol; benzodioxola; metilenodioxi; CYP450; bióforo; compostos bioativos. Abstract Safrole (1), obtained from essential oils from different plant species, has wide application in the chemical industry as a synthetic precursor of piperonyl butoxide, piperonal and drugs such as tadalafil, cinoxacin and levodopa. From the toxicological point of view, it is considered a hepatotoxin through metabolic bioactivation, leading to the formation of electrophilic metabolites, and has been described as an inhibitor of different CYP450 isoenzymes. The versatility of its structure, allowing various chemical transformations, and the biophoric nature of its benzodioxole or methylenedioxy subunit, give it unique features and make it an attractive starting material for the synthesis of compounds with different pharmacological activities. -
THE THIOBARBITURIC ACID TEST in IRRADIATION-STERILIZED BEEF By
THE THIOBARBITURIC ACID TEST IN IRRADIATION-STERILIZED BEEF by NORMAN LEE SMITH A THESIS submitted to OREGON STATE COLLEGE in partial fulfillment of the requirements for the degree of MASTER OF SCIENCE June 1959 APPROVED: Redacted for Privacy Associate Professor of Chemistry In Ghare of Major Redacted for Privacy Chairman of Department of Chemistry Redacted for Prîvacy Chairman of choo1 Graduate Committee Dean of Graduate School Date thesi9 19 presented July 10, 195B Typed by Cliatie Stoddard AC KOV'LEDGEMENTS The author would like to exprese his most sincere appreciation to Dr. E. C. Bubi, who by his guidance, help, and enthusiasm has made work on this project both an education and a pleasure. Especial thanks also o to Dr. Ian J. Tinsley, whose unfailing supply of timely suggestions, il1uminatin dis- cussions, and refreshing good humor was most generously shared. TABLE OF CONTENTS Chapter pase i INTRODUC T ION . i 2. LITERATURE STJRVEY . 5 3. PREPARATION AND IRRADIATION OF SAMPLES . 10 4. COLOR TESTS WITH THIOBARBITURIC ACID . 12 5. REAGENTS AND INSTRUMENTS . 15 6. GENERAL INFORMATION ON THE MEAT-TBA REACTION 17 Meat fractions and TBA reaction . 17 Spectral curves of meat-TBA piment solutions . 21 Autoc1avin of meat sampleß . ¿5 7. GENERAL INFORMATION ON THE GLYOXAL-TEA flc..tt'si.Lmr\?s1 L)i s S S S I S S S S ' Effect of pli on glyoxal-TBA reaction . 26 Glyoxal-barbituric acid reaction . 26 Barbituric acid reaction with beef . , 27 para-aminoacetophenone reaction with 1yoxal, malonaldehyde, and irradiated beef . 27 8. GLYOXAL-TBA AND :EAT-TBA PIGMENT PJI- FICATION e s . -
Metabolic Carbonyl Reduction of Anthracyclines — Role in Cardiotoxicity and Cancer Resistance
Invest New Drugs DOI 10.1007/s10637-017-0443-2 REVIEW Metabolic carbonyl reduction of anthracyclines — role in cardiotoxicity and cancer resistance. Reducing enzymes as putative targets for novel cardioprotective and chemosensitizing agents Kamil Piska1 & Paulina Koczurkiewicz1 & Adam Bucki 2 & Katarzyna Wójcik-Pszczoła1 & Marcin Kołaczkowski2 & Elżbieta Pękala1 Received: 23 November 2016 /Accepted: 17 February 2017 # The Author(s) 2017. This article is published with open access at Springerlink.com Summary Anthracycline antibiotics (ANT), such as doxoru- monoHER, curcumin, (−)-epigallocatechin gallate, resvera- bicin or daunorubicin, are a class of anticancer drugs that are trol, berberine or pixantrone, and their modulating effect on widely used in oncology. Although highly effective in cancer the activity of ANT is characterized and discussed as potential therapy, their usefulness is greatly limited by their mechanism of action for novel therapeutics in cancer cardiotoxicity. Possible mechanisms of ANT cardiotoxicity treatment. include their conversion to secondary alcohol metabolites (i.e. doxorubicinol, daunorubicinol) catalyzed by carbonyl re- Keywords Anthracyclines . Cardiotoxicity . Resistance . ductases (CBR) and aldo-keto reductases (AKR). These me- Pharmacokinetics . Drug metabolism . Anticancer agents tabolites are suspected to be more cardiotoxic than their parent compounds. Moreover, overexpression of ANT-reducing en- zymes (CBR and AKR) are found in many ANT-resistant Introduction cancers. The secondary metabolites show decreased cytotoxic properties and are more susceptible to ABC-mediated efflux Anthracyclines (ANT) are a class of cell-cycle non-specific than their parent compounds; thus, metabolite formation is anticancer antibiotics that were first isolated from the considered one of the mechanisms of cancer resistance. Streptomyces genus in the early 1960s. -
WO 2016/103058 Al 30 June 2016 (30.06.2016) P O P C T
(12) INTERNATIONAL APPLICATION PUBLISHED UNDER THE PATENT COOPERATION TREATY (PCT) (19) World Intellectual Property Organization I International Bureau (10) International Publication Number (43) International Publication Date WO 2016/103058 Al 30 June 2016 (30.06.2016) P O P C T (51) International Patent Classification: (74) Agent: KHURANA & KHURANA, ADVOCATES & IP C07D 317/68 (2006.01) C07C 45/56 (2006.01) ATTORNEYS; E-13, UPSIDC, Site-IV, Behind-Grand Venice, Kasna Road, UP, National Capital Region, Greater (21) International Application Number: Noida 201310 (IN). PCT/IB201 5/053 112 (81) Designated States (unless otherwise indicated, for every (22) International Filing Date: kind of national protection available): AE, AG, AL, AM, 29 April 2015 (29.04.2015) AO, AT, AU, AZ, BA, BB, BG, BH, BN, BR, BW, BY, (25) Filing Language: English BZ, CA, CH, CL, CN, CO, CR, CU, CZ, DE, DK, DM, DO, DZ, EC, EE, EG, ES, FI, GB, GD, GE, GH, GM, GT, (26) Publication Language: English HN, HR, HU, ID, IL, IN, IR, IS, JP, KE, KG, KN, KP, KR, (30) Priority Data: KZ, LA, LC, LK, LR, LS, LU, LY, MA, MD, ME, MG, 4124/MUM/2014 23 December 2014 (23. 12.2014) IN MK, MN, MW, MX, MY, MZ, NA, NG, NI, NO, NZ, OM, PA, PE, PG, PH, PL, PT, QA, RO, RS, RU, RW, SA, SC, (71) Applicant: ANTHEA AROMATIC S PRIVATE LIM¬ SD, SE, SG, SK, SL, SM, ST, SV, SY, TH, TJ, TM, TN, ITED [IN/IN]; R-81/82 TTC Industrial Area, Rabale Midc, TR, TT, TZ, UA, UG, US, UZ, VC, VN, ZA, ZM, ZW. -
Atmospheric Fate of a Series of Methyl Saturated Alcohols
https://doi.org/10.5194/acp-2019-662 Preprint. Discussion started: 9 August 2019 c Author(s) 2019. CC BY 4.0 License. 1 Atmospheric fate of a series of Methyl Saturated Alcohols 2 (MSA): Kinetic and Mechanistic study 3 Inmaculada Colmenar1,2, Pilar Martin1,2, Beatriz Cabañas1,2, Sagrario Salgado1,2, Araceli 4 Tapia1,2, Inmaculada Aranda1,2 5 1Universidad de Castilla La Mancha, Departamento de Química Física, Facultad de Ciencias y Tecnologías 6 Químicas, Avda. Camilo José Cela S/N, 13071 Ciudad Real, Spain 7 2Universidad de Castilla La Mancha, Instituto de Combustión y Contaminación Atmosférica (ICCA), Camino 8 Moledores S/N, 13071 Ciudad Real, Spain 9 Correspondence to: Pilar Martín ([email protected]) 10 Abstract. The atmospheric fate of a series of Methyl Saturated Alcohols (MSA) has been evaluated through the 11 kinetic and reaction product studies with the main atmospheric oxidants. Rate coefficients (in cm3 molecule-1 s-1 12 unit) measured at 298K and atmospheric pressure ( 740 Torr) were as follows: (3.71 ± 0.53) × 10-10, (1.91 ± -11 -15 13 0.65) × 10 and (2.92 ± 1.38) × 10 for reaction of E-4-methyl-cyclohexanol with Cl, OH and NO3, respectively. 14 (2.70 ± 0.55) × 10-10 and (5.57 ± 0.66) × 10-12 for reaction of 3,3-dimethyl-1-butanol with Cl and OH radical 15 respectively and (1.21 ± 0.37) × 10-10 and (10.51 ± 0.81) × 10-12 for reaction of 3,3-dimethyl-2-butanol with Cl 16 and OH radical respectively. The main detected products were 4-methylcyclohexanone, 3,3-dimethylbutanal and 17 3,3-dimethyl-2-butanone for the reactions of E-4-methyl-cyclohexanol, 3,3-dimethyl-1-butanol and 3,3-dimethyl- 18 2-butanol respectively with the three oxidants. -
Feni Nanoparticles with Carbon Armor As Sustainable Hydrogenation Catalysts: Towards Cite This: J
Journal of Materials Chemistry A View Article Online COMMUNICATION View Journal | View Issue FeNi nanoparticles with carbon armor as sustainable hydrogenation catalysts: towards Cite this: J. Mater. Chem. A,2014,2, 11591 biorefineries† Received 15th May 2014 Gianpaolo Chieffi, Cristina Giordano, Markus Antonietti and Davide Esposito* Accepted 28th May 2014 DOI: 10.1039/c4ta02457e www.rsc.org/MaterialsA Carbon supported FeNi nanoparticles were prepared by carbothermal and electronic properties of the active sites. Moreover, catalyst reduction of cellulose filter paper impregnated with Fe and Ni salts. stability is improved by inhibiting the sintering or the leaching The resulting carbon enwrapped alloy nanoparticles were employed of the active species through interaction with the second metal. as an efficient catalyst for the continuous hydrogenation of molecules The development of new sustainable hydrogenation catalysts Creative Commons Attribution 3.0 Unported Licence. obtainable from different fractions of lignocellulosic biomass. Scale- with superior stability is also highly desired for the successful up and time on stream tests over 80 hours proved the catalyst stable development of bioreneries for the conversion of raw ligno- and durable of over a wide range of conditions. cellulosic materials into chemicals and fuels, where poor selectivity and catalyst deactivation are sensibly affected by the high heterogeneity of biomass. In the present work we describe the sustainable synthesis of Introduction a carbonized lter paper (CFP) supported bimetallic FeNi alloy catalyst and investigate its activity during the continuous Catalytic hydrogenation has been one of the most studied hydrogenation of different functional groups and model This article is licensed under a reactions for decades. -
Glyoxylic Acid. by VICTORJOHN HARDING and CHARLESWEIZMANN
View Article Online / Journal Homepage / Table of Contents for this issue 1126 HARDlNC3 AND WEIZMANN : SYNTHESIS OF XCIV.-Synthesis of 6-Carboxy-3: 4-dimethoxyphenyl- glyoxylic Acid. By VICTORJOHN HARDING and CHARLESWEIZMANN. AMONGthe products of the oxidation of trimethylbrazilin by means of warm potassium permanganate, there was found to occur a dibasic acid possessing the empirical formula C,,H,,O,. This acid, which was isolated and investigated in these laboratories by W. H. Perkin, jun., was shown to be 6-carboxy-3: 4-dimethoxy- phenylglyoxylic acid (I) [“ dimethoxycarboxybenzoylformic acid ”1 (Trans., 1902, 81, 1022). In the present communication the authors describe the synthesis of this acid from 4 : 5-dimethoxy-o-methyZacetophenone(11) : MeO/\C02H MeOAMe MeO/\Me MeOl ‘CO*C02H MeOI ICOMe Me01 /CO*CO,H \/ \/ \/ (1.1 (11.) (111.) This ketone is easily prepared by the condensation of acetyl chloride and homocatechol dimethyl ether by means of aluminium chloride. When oxidised by warm potassium permanganate there is first isolated 4 : 5-clinz,ethoxy-o-tolylgl~o~l~cacid (111). Further oxidation converts the methyl group into a carboxy-group, and leads to the production of 6-carboxy-3 : 4-dimethoxyphenylglyoxylic acid. Although it has not been found possible directly to compare the synthetical acid with that obtained from trimethylbrazilin, the Published on 01 January 1910. Downloaded by New York University 27/02/2016 21:27:57. authors do not think there can be much doubt as to their identity. The following are the properties of the synthetical acid: 1. Calcium cE’loride, with a neutral solution of the ammonium salt, gives no precipitate in the cold, but on warming, the crystalline calcium salt separates af; once. -
Thermodynamic Constraints Shape the Structure of Carbon Fixation Pathways
Biochimica et Biophysica Acta 1817 (2012) 1646–1659 Contents lists available at SciVerse ScienceDirect Biochimica et Biophysica Acta journal homepage: www.elsevier.com/locate/bbabio Thermodynamic constraints shape the structure of carbon fixation pathways Arren Bar-Even, Avi Flamholz, Elad Noor, Ron Milo ⁎ Department of Plant Sciences, The Weizmann Institute of Science, Rehovot 76100, Israel article info abstract Article history: Thermodynamics impose a major constraint on the structure of metabolic pathways. Here, we use carbon fixa- Received 27 March 2012 tion pathways to demonstrate how thermodynamics shape the structure of pathways and determine the cellular Received in revised form 7 May 2012 resources they consume. We analyze the energetic profile of prototypical reactions and show that each reaction Accepted 9 May 2012 type displays a characteristic change in Gibbs energy. Specifically, although carbon fixation pathways display a Available online 17 May 2012 considerable structural variability, they are all energetically constrained by two types of reactions: carboxylation and carboxyl reduction. In fact, all adenosine triphosphate (ATP) molecules consumed by carbon fixation path- Keywords: – – Reduction potential ways with a single exception are used, directly or indirectly, to power one of these unfavorable reactions. Carbon fixation When an indirect coupling is employed, the energy released by ATP hydrolysis is used to establish another chem- Thermodynamic favorability ical bond with high energy of hydrolysis, e.g. a thioester. This bond is cleaved by a downstream enzyme to ener- Exergonism gize an unfavorable reaction. Notably, many pathways exhibit reduced ATP requirement as they couple Reaction coupling unfavorable carboxylation or carboxyl reduction reactions to exergonic reactions other than ATP hydrolysis. -
Safety Data Sheet
SAFETY DATA SHEET Revision Date 14-Feb-2020 Revision Number 2 1. Identification Product Name 3,3-Dimethyl-2-butanol Cat No. : 43725 CAS-No 464-07-3 Synonyms Pinacolyl Alcohol.; Tert-Butyl Methyl Carbinol Recommended Use Laboratory chemicals. Uses advised against Food, drug, pesticide or biocidal product use. Details of the supplier of the safety data sheet Company Alfa Aesar Thermo Fisher Scientific Chemicals, Inc. 30 Bond Street Ward Hill, MA 01835-8099 Tel: 800-343-0660 Fax: 800-322-4757 Email: [email protected] www.alfa.com Emergency Telephone Number During normal business hours (Monday-Friday, 8am-7pm EST), call (800) 343-0660. After normal business hours, call Carechem 24 at (866) 928-0789. 2. Hazard(s) identification Classification This chemical is considered hazardous by the 2012 OSHA Hazard Communication Standard (29 CFR 1910.1200) Flammable liquids Category 3 Label Elements Signal Word Warning Hazard Statements Flammable liquid and vapor ______________________________________________________________________________________________ Page 1 / 7 3,3-Dimethyl-2-butanol Revision Date 14-Feb-2020 ______________________________________________________________________________________________ Precautionary Statements Prevention Keep away from heat/sparks/open flames/hot surfaces. - No smoking Keep container tightly closed Ground/bond container and receiving equipment Use explosion-proof electrical/ventilating/lighting/equipment Use only non-sparking tools Take precautionary measures against static discharge Wear protective gloves/protective clothing/eye protection/face protection Skin IF ON SKIN (or hair): Take off immediately all contaminated clothing. Rinse skin with water/shower Fire In case of fire: Use CO2, dry chemical, or foam for extinction Storage Store in a well-ventilated place. Keep cool Disposal Dispose of contents/container to an approved waste disposal plant Hazards not otherwise classified (HNOC) None identified 3.