Whole-Brain Mapping of Monosynaptic Inputs to Midbrain
Total Page:16
File Type:pdf, Size:1020Kb
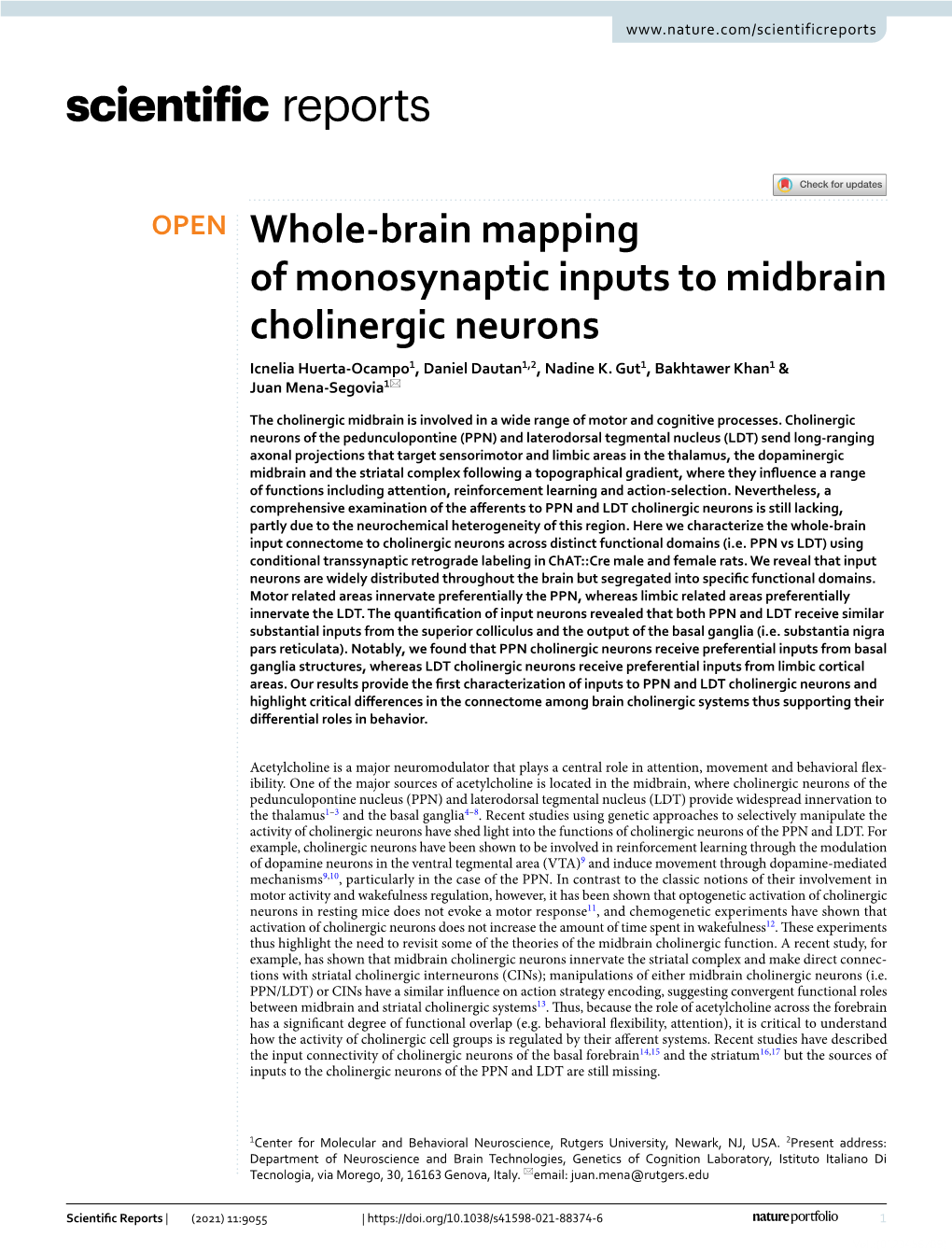
Load more
Recommended publications
-
Synaptic Organization of Claustral and Geniculate Afferents to the Visual Cortex of the Cat
The Journal of Neuroscience December 1986, 6(12): 3564-3575 Synaptic Organization of Claustral and Geniculate Afferents to the Visual Cortex of the Cat Simon LeVay Robert Bosch Vision Research Center, Salk Institute for Biological Studies, San Diego, California 92138 Claustral and geniculate afferents to area 17 were labeled by sponses of some cortical neurons, previously called “hypercom- anterograde axonal transport of peroxidase-conjugated wheat- plex cells” by Hubel and Wiesel (1965), are suppressed as the germ agglutinin, and examined in the electron microscope. A length of the stimulating slit of light is extended beyond some peroxidase reaction protocol that led to labeling in the form of optimal value. Interestingly, neurons in the visual claustrum minute holes in the EM sections was used. Both types of affer- behave in a complementary fashion: Their responses to an ori- ents formed type 1 (presumed excitatory) synapses exclusively. ented stimulus increase monotonically with stimulus length, In agreement with previous reports the great majority of genic- sometimes showing length summation up to 40” or more-a ulate afferents to layers 4 and 6 contacted dendritic spines. The sizable portion of the animal’s entire field of view (Sherk and claustral afferents to layers 1 and 6 also predominantly con- LeVay, 198 1). tacted spines. In layer 4, however, claustral afferents contacted These observations suggest that claustral neurons contribute spines and dendritic shafts about equally. The results suggest a to end-stopping by exerting a length-dependent inhibition on substantial Claus&al input to smooth-dendrite cells in layer 4, some neurons in the visual cortex. -
Circuits in the Rodent Brainstem That Control Whisking in Concert with Other Orofacial Motor Actions
Neuroscience 368 (2018) 152–170 CIRCUITS IN THE RODENT BRAINSTEM THAT CONTROL WHISKING IN CONCERT WITH OTHER OROFACIAL MOTOR ACTIONS y y LAUREN E. MCELVAIN, a BETH FRIEDMAN, a provides the reset to the relevant premotor oscillators. HARVEY J. KARTEN, b KAREL SVOBODA, c FAN WANG, d Third, direct feedback from somatosensory trigeminal e a,f,g MARTIN DESCHEˆ NES AND DAVID KLEINFELD * nuclei can rapidly alter motion of the sensors. This feed- a Department of Physics, University of California at San Diego, back is disynaptic and can be tuned by high-level inputs. La Jolla, CA 92093, USA A holistic model for the coordination of orofacial motor actions into behaviors will encompass feedback pathways b Department of Neurosciences, University of California at San Diego School of Medicine, La Jolla, CA 92093, USA through the midbrain and forebrain, as well as hindbrain c areas. Howard Hughes Medical Institute, Janelia Research This article is part of a Special Issue entitled: Barrel Campus, Ashburn, VA 20147, USA Cortex. Ó 2017 IBRO. Published by Elsevier Ltd. All rights d Department of Neurobiology, Duke University Medical reserved. Center, Durham, NC 27710, USA e Department of Psychiatry and Neuroscience, Laval University, Que´bec City, G1J 2G3, Canada f Key words: coupled oscillators, facial nucleus, hypoglossal Section of Neurobiology, University of California at San Diego, La Jolla, CA 92093, USA nucleus, licking, orienting, tongue, vibrissa. g Department of Electrical and Computer Engineering, University of California at San Diego, La Jolla, CA 92093, USA Contents Introduction 153 Abstract—The world view of rodents is largely determined Coordination of multiple orofacial motor actions 153 by sensation on two length scales. -
Basal Ganglia Anatomy, Physiology, and Function Ns201c
Basal Ganglia Anatomy, Physiology, and Function NS201c Human Basal Ganglia Anatomy Basal Ganglia Circuits: The ‘Classical’ Model of Direct and Indirect Pathway Function Motor Cortex Premotor Cortex + Glutamate Striatum GPe GPi/SNr Dopamine + - GABA - Motor Thalamus SNc STN Analagous rodent basal ganglia nuclei Gross anatomy of the striatum: gateway to the basal ganglia rodent Dorsomedial striatum: -Inputs predominantly from mPFC, thalamus, VTA Dorsolateral striatum: -Inputs from sensorimotor cortex, thalamus, SNc Ventral striatum: Striatal subregions: Dorsomedial (caudate) -Inputs from vPFC, hippocampus, amygdala, Dorsolateral (putamen) thalamus, VTA Ventral (nucleus accumbens) Gross anatomy of the striatum: patch and matrix compartments Patch/Striosome: -substance P -mu-opioid receptor Matrix: -ChAT and AChE -somatostatin Microanatomy of the striatum: cell types Projection neurons: MSN: medium spiny neuron (GABA) •striatonigral projecting – ‘direct pathway’ •striatopallidal projecting – ‘indirect pathway’ Interneurons: FS: fast-spiking interneuron (GABA) LTS: low-threshold spiking interneuron (GABA) LA: large aspiny neuron (ACh) 30 um Cellular properties of striatal neurons Microanatomy of the striatum: striatal microcircuits • Feedforward inhibition (mediated by fast-spiking interneurons) • Lateral feedback inhibition (mediated by MSN collaterals) Basal Ganglia Circuits: The ‘Classical’ Model of Direct and Indirect Pathway Function Motor Cortex Premotor Cortex + Glutamate Striatum GPe GPi/SNr Dopamine + - GABA - Motor Thalamus SNc STN The simplified ‘classical’ model of basal ganglia circuit function • Information encoded as firing rate • Basal ganglia circuit is linear and unidirectional • Dopamine exerts opposing effects on direct and indirect pathway MSNs Basal ganglia motor circuit: direct pathway Motor Cortex Premotor Cortex Glutamate Striatum GPe GPi/SNr Dopamine + GABA Motor Thalamus SNc STN Direct pathway MSNs express: D1, M4 receptors, Sub. -
Conditional Ablation of Brain-Derived Neurotrophic Factor-Trkb Signaling Impairs Striatal Neuron Development
Conditional ablation of brain-derived neurotrophic factor-TrkB signaling impairs striatal neuron development Yun Lia,1, Daishi Yuia, Bryan W. Luikarta,2, Renée M. McKaya, Yanjiao Lia, John L. Rubensteinb, and Luis F. Paradaa,3 aDepartment of Developmental Biology and Kent Waldrep Center for Basic Research on Nerve Growth and Regeneration, University of Texas Southwestern Medical Center, Dallas, TX 75390; and bNina Ireland Laboratory of Developmental Neurobiology, Department of Psychiatry, University of California, San Francisco, CA 94143 Contributed by Luis F. Parada, August 2, 2012 (sent for review June 21, 2012) Neurotrophic factors, such as brain-derived neurotrophic factor cascades pertaining to development, maturation, and function of (BDNF), are associated with the physiology of the striatum and the striatum remains to be delineated. the loss of its normal functioning under pathological conditions. In this study, we conditionally ablated BDNF or its receptor The role of BDNF and its downstream signaling in regulating the TrkB in corticostriatal and nigrostriatal neuronal circuits. We development of the striatum has not been fully investigated, found that Bdnf deletion in both cortex and substantia nigra led to Bdnf complete depletion of BDNF protein in the striatum. Mutant mice however. Here we report that ablation of in both the cortex displayed dramatic developmental abnormalities and neurological and substantia nigra depletes BDNF in the striatum, and leads to impairments. Furthermore, specific deletion of TrkB from striatal fi impaired striatal development, severe motor de cits, and postnatal neurons was sufficient to produce this wide range of developmental lethality. Furthermore, striatal-specific ablation of TrkB, the gene deficits. Thus, our results demonstrate that BDNF and TrkB play encoding the high-affinity receptor for BDNF, is sufficient to elicit critical paracrine and cell-autonomous roles, respectively, in the an array of striatal developmental abnormalities, including de- development and maintenance of striatal neurons. -
Electrophysiological and Morphological Evidence for a Gabaergic Nigrostriatal Pathway
The Journal of Neuroscience, June 1, 1999, 19(11):4682–4694 Electrophysiological and Morphological Evidence for a GABAergic Nigrostriatal Pathway Manuel Rodrı´guez1 and Toma´ s Gonza´ lez-Herna´ ndez2 Departments of 1Physiology and 2Anatomy, Faculty of Medicine, University of La Laguna, La Laguna, Tenerife, Canary Islands, Spain The electrophysiological and neurochemical characteristics of gic neurons, a percentage that reached 81–84% after 6-OHDA the nondopaminergic nigrostriatal (NO-DA) cells and their func- injection. Electrophysiologically, NO-DA cells showed a behavior tional response to the degeneration of dopaminergic nigrostri- similar to that found in other nigral GABAergic (nigrothalamic) atal (DA) cells were studied. Three different criteria were used to cells. In addition, the 6-OHDA degeneration of DA cells induced a identify NO-DA cells: (1) antidromic response to striatal stimu- modification of their electrophysiological pattern similar to that lation with an electrophysiological behavior (firing rate, inter- found in GABAergic nigrothalamic neurons. Taken together, the spike interval variability, and conduction velocity) different from present data indicate the existence of a small GABAergic nigro- that of DA cells; (2) retrograde labeling after striatal injection of striatal pathway and suggest their involvement in the pathophys- HRP but showing immunonegativity for DA cell markers (ty- iology of Parkinson’s disease. rosine hydroxylase, calretinin, calbindin-D28k, and cholecysto- kinin); and (3) resistance to neurotoxic effect of 6-hydroxydomine Key words: nigrostriatal pathway; dopaminergic cells; (6-OHDA). Our results showed that under normal conditions, GABAergic cells; GABA; glutamic acid decarboxylase; parval- 5–8% of nigrostriatal neurons are immunoreactive for GABA, glu- bumin; calretinin; calbindin-D28k; cholecystokinin; Parkinson’s tamic acid decarboxylase, and parvalbumin, markers of GABAer- disease The substantia nigra (SN) is a major output center of the basal paminergic (Grace and Bunney, 1980, 1983a). -
Injection of a Dopamine Type 2 Receptor Antagonist Into the Dorsal Striatum Disrupts Choices Driven by Previous Outcomes, but Not Perceptual Inference
6298 • The Journal of Neuroscience, April 22, 2015 • 35(16):6298–6306 Behavioral/Cognitive Injection of a Dopamine Type 2 Receptor Antagonist into the Dorsal Striatum Disrupts Choices Driven by Previous Outcomes, But Not Perceptual Inference X Eunjeong Lee, Moonsang Seo, Olga Dal Monte, and Bruno B. Averbeck Laboratory of Neuropsychology, National Institute of Mental Health, National Institutes of Health, Bethesda, Maryland 20892-4415 Decisions are often driven by a combination of immediate perception and previous experience. In this study, we investigated how these two sources of information are integrated and the neural systems that mediate this process. Specifically, we injected a dopamine type 1 antagonist (D1A; SCH23390) or a dopamine type 2 antagonist (D2A; eticlopride) into the dorsal striatum while macaques performed a task in which their choices were driven by perceptual inference and/or reinforcement of past choices. We found that the D2A affected choices based on previous outcomes. However, there were no effects of the D2A on choices driven by perceptual inference. We found that the D1A did not affect perceptual inference or reinforcement learning. Finally, a Bayesian model applied to the results suggested that the D2Amaybeincreasingnoiseinthestriatalrepresentationofvalue,perhapsbydisruptingthestriatalpopulationthatnormallyrepresents value. Key words: action value; dorsal striatum; neuromodulation; Parkinson’s disease; reinforcement learning; sequential decision making Introduction available in the current trial to drive the choice. Reinforcement Decisions are often driven by a combination of immediate per- learning or learning from past outcomes has often been attrib- ceptual evidence and previous experience. Several groups have uted to plasticity within the striatum (Graybiel, 2008; Cockburn studied perceptual decision-making tasks, in which stochastic et al., 2014). -
Age-Related Change in 5-HT6 Receptor Availability in Healthy Male Volunteers Measured with 11C-GSK215083 PET
BRIEF COMMUNICATION Age-Related Change in 5-HT6 Receptor Availability in Healthy Male Volunteers Measured with 11C-GSK215083 PET Rajiv Radhakrishnan1, Nabeel Nabulsi2, Edward Gaiser1, Jean-Dominique Gallezot2, Shannan Henry2, Beata Planeta2, Shu-fei Lin2, Jim Ropchan2, Wendol Williams1, Evan Morris2,3, Deepak Cyril D’Souza1, Yiyun Huang2, Richard E. Carson2,3, and David Matuskey1,2 1Department of Psychiatry, Yale University School of Medicine, New Haven, Connecticut; 2Department of Radiology and Biomedical Imaging, Yale University School of Medicine, New Haven, Connecticut; and 3Department of Biomedical Engineering, Yale University, New Haven, Connecticut because it presents an attractive therapeutic target for neuropsychi- Serotonin receptor 6 (5-hydroxytrypamine-6, or 5-HT6) is a potential atric disorders. therapeutic target given its distribution in brain regions that are Functionally, 5-HT6 exhibits excitatory action, but it can also important in depression, anxiety, and cognition. This study sought colocalize with g-aminobutyric acid–ergic neurons and produce an to investigate the effects of age on 5-HT6 receptor availability using inhibition of brain activity leading to complicated and discrepant 11 C-GSK215083, a PET ligand with affinity for 5-HT6 in the striatum results (2,4). Heterogeneous effects are also seen with other neu- and 5-HT in the cortex. Methods: Twenty-eight healthy male vol- 2A rotransmitters in specific brain regions, with 5-HT antagonism unteers (age range, 23–52 y) were scanned with 11C-GSK215083 6 PET. Time–activity curves in regions of interest were fitted using a resulting in increased extracellular glutamate, dopamine, and ace- multilinear analysis method. Nondisplaceable binding potential tylcholine in the frontal cortex and hippocampus (5,6), whereas (BPND) was calculated using the cerebellum as the reference region 5-HT6 agonists have been shown to produce increased extracellu- and corrected for partial-volume effects. -
Prominent Activation of Brainstem and Pallidal Afferents of the Ventral Tegmental Area by Cocaine
Neuropsychopharmacology (2008) 33, 2688–2700 & 2008 Nature Publishing Group All rights reserved 0893-133X/08 $30.00 www.neuropsychopharmacology.org Prominent Activation of Brainstem and Pallidal Afferents of the Ventral Tegmental Area by Cocaine 1,3 2 1 1 1 Stefanie Geisler , Michela Marinelli , Beth DeGarmo , Mary L Becker , Alexander J Freiman , 2 2 ,1 Mitch Beales , Gloria E Meredith and Daniel S Zahm* 1 2 Department of Pharmacological and Physiological Science, Saint Louis University School of Medicine, St Louis, MO, USA; Department of Cellular & Molecular Pharmacology, Rosalind Franklin University of Medicine and Science, North Chicago, IL, USA Blockade of monoamine transporters by cocaine should not necessarily lead to certain observed consequences of cocaine administration, including increased firing of ventral mesencephalic dopamine (DA) neurons and accompanying impulse-stimulated release of DA in the forebrain and cortex. Accordingly, we hypothesize that the dopaminergic-activating effect of cocaine requires stimulation of the dopaminergic neurons by afferents of the ventral tegmental area (VTA). We sought to determine if afferents of the VTA are activated following cocaine administration. Rats were injected in the VTA with retrogradely transported Fluoro-Gold and, after 1 week, were allowed to self-administer cocaine or saline via jugular catheters for 2 h on 6 consecutive days. Other rats received a similar amount of investigator- administered cocaine through jugular catheters. Afterward, the rats were killed and the brains processed immunohistochemically for retrogradely transported tracer and Fos, the protein product of the neuronal activation-associated immediate early gene, c-fos. Forebrain neurons exhibiting both Fos and tracer immunoreactivity were enriched in both cocaine groups relative to the controls only in the globus pallidus and ventral pallidum, which, together, represented a minor part of total forebrain retrogradely labeled neurons. -
The Effects of Chloride Dynamics on Substantia Nigra Pars Reticulata
RESEARCH ARTICLE The effects of chloride dynamics on substantia nigra pars reticulata responses to pallidal and striatal inputs Ryan S Phillips1,2, Ian Rosner2,3, Aryn H Gittis2,3, Jonathan E Rubin1,2* 1Department of Mathematics, University of Pittsburgh, Pittsburgh, United States; 2Center for the Neural Basis of Cognition, Pittsburgh, United States; 3Department of Biological Sciences, Carnegie Mellon University, Pittsburgh, United States Abstract As a rodent basal ganglia (BG) output nucleus, the substantia nigra pars reticulata (SNr) is well positioned to impact behavior. SNr neurons receive GABAergic inputs from the striatum (direct pathway) and globus pallidus (GPe, indirect pathway). Dominant theories of action selection rely on these pathways’ inhibitory actions. Yet, experimental results on SNr responses to these inputs are limited and include excitatory effects. Our study combines experimental and computational work to characterize, explain, and make predictions about these pathways. We observe diverse SNr responses to stimulation of SNr-projecting striatal and GPe neurons, including biphasic and excitatory effects, which our modeling shows can be explained by intracellular chloride processing. Our work predicts that ongoing GPe activity could tune the SNr operating mode, including its responses in decision-making scenarios, and GPe output may modulate synchrony and low-frequency oscillations of SNr neurons, which we confirm using optogenetic stimulation of GPe terminals within the SNr. Introduction The substantia nigra pars reticulata (SNr) is the primary output nucleus of the rodent basal ganglia *For correspondence: (BG) and hence likely plays a key role in the behavioral functions, such as decision-making and action [email protected] selection, suppression, or tuning, to which the BG contribute. -
Mapping the Populations of Neurotensin Neurons in the Male Mouse Brain T Laura E
Neuropeptides 76 (2019) 101930 Contents lists available at ScienceDirect Neuropeptides journal homepage: www.elsevier.com/locate/npep Mapping the populations of neurotensin neurons in the male mouse brain T Laura E. Schroeder, Ryan Furdock, Cristina Rivera Quiles, Gizem Kurt, Patricia Perez-Bonilla, ⁎ Angela Garcia, Crystal Colon-Ortiz, Juliette Brown, Raluca Bugescu, Gina M. Leinninger Department of Physiology, Michigan State University, East Lansing, MI 48114, United States ARTICLE INFO ABSTRACT Keywords: Neurotensin (Nts) is a neuropeptide implicated in the regulation of many facets of physiology, including car- Lateral hypothalamus diovascular tone, pain processing, ingestive behaviors, locomotor drive, sleep, addiction and social behaviors. Parabrachial nucleus Yet, there is incomplete understanding about how the various populations of Nts neurons distributed throughout Periaqueductal gray the brain mediate such physiology. This knowledge gap largely stemmed from the inability to simultaneously Central amygdala identify Nts cell bodies and manipulate them in vivo. One means of overcoming this obstacle is to study NtsCre Thalamus mice crossed onto a Cre-inducible green fluorescent reporter line (NtsCre;GFP mice), as these mice permit both Nucleus accumbens Preoptic area visualization and in vivo modulation of specific populations of Nts neurons (using Cre-inducible viral and genetic tools) to reveal their function. Here we provide a comprehensive characterization of the distribution and relative Abbreviation: 12 N, Hypoglossal nucleus; -
Circuit Architecture of VTA Dopamine Neurons Revealed by Systematic Input-Output Mapping
Article Circuit Architecture of VTA Dopamine Neurons Revealed by Systematic Input-Output Mapping Graphical Abstract Authors Kevin T. Beier, Elizabeth E. Steinberg, Katherine E. DeLoach, ..., Eric J. Kremer, Robert C. Malenka, Liqun Luo Correspondence [email protected] (R.C.M.), [email protected] (L.L.) In Brief A combination of state-of-the-art viral- genetic tools shows that dopaminergic neurons in the ventral tegmental area (VTA-DA) employ biased-input/discrete- output circuit architecture, allowing the construction of an input-output map for further investigation of the neural circuits underlying the different functions of these neurons in psychological processes and brain diseases. Highlights d VTA dopamine (DA) and GABA neurons receive similar inputs from diverse sources d VTA-DA neurons projecting to different output sites receive biased input d VTA-DA neurons projecting to lateral and medial NAc innervate non-overlapping targets d A top-down anterior cortex/VTA-DA/lateral NAc circuit is reinforcing Beier et al., 2015, Cell 162, 622–634 July 30, 2015 ª2015 Elsevier Inc. http://dx.doi.org/10.1016/j.cell.2015.07.015 Article Circuit Architecture of VTA Dopamine Neurons Revealed by Systematic Input-Output Mapping Kevin T. Beier,1,2 Elizabeth E. Steinberg,2 Katherine E. DeLoach,1 Stanley Xie,1 Kazunari Miyamichi,1,5 Lindsay Schwarz,1 Xiaojing J. Gao,1,6 Eric J. Kremer,3,4 Robert C. Malenka,2,* and Liqun Luo1,* 1Howard Hughes Medical Institute and Department of Biology, Stanford University, Stanford, CA 94305, USA 2Nancy Pritzker Laboratory, -
Distribution of Dopamine D3 Receptor Expressing Neurons in the Human Forebrain: Comparison with D2 Receptor Expressing Neurons Eugenia V
Distribution of Dopamine D3 Receptor Expressing Neurons in the Human Forebrain: Comparison with D2 Receptor Expressing Neurons Eugenia V. Gurevich, Ph.D., and Jeffrey N. Joyce, Ph.D. The dopamine D2 and D3 receptors are members of the D2 important difference from the rat is that D3 receptors were subfamily that includes the D2, D3 and D4 receptor. In the virtually absent in the ventral tegmental area. D3 receptor rat, the D3 receptor exhibits a distribution restricted to and D3 mRNA positive neurons were observed in sensory, mesolimbic regions with little overlap with the D2 receptor. hormonal, and association regions such as the nucleus Receptor binding and nonisotopic in situ hybridization basalis, anteroventral, mediodorsal, and geniculate nuclei of were used to study the distribution of the D3 receptors and the thalamus, mammillary nuclei, the basolateral, neurons positive for D3 mRNA in comparison to the D2 basomedial, and cortical nuclei of the amygdala. As revealed receptor/mRNA in subcortical regions of the human brain. by simultaneous labeling for D3 and D2 mRNA, D3 mRNA D2 binding sites were detected in all brain areas studied, was often expressed in D2 mRNA positive neurons. with the highest concentration found in the striatum Neurons that solely expressed D2 mRNA were numerous followed by the nucleus accumbens, external segment of the and regionally widespread, whereas only occasional D3- globus pallidus, substantia nigra and ventral tegmental positive-D2-negative cells were observed. The regions of area, medial preoptic area and tuberomammillary nucleus relatively higher expression of the D3 receptor and its of the hypothalamus. In most areas the presence of D2 mRNA appeared linked through functional circuits, but receptor sites coincided with the presence of neurons co-expression of D2 and D3 mRNA suggests a functional positive for its mRNA.