Using Signals of Opportunity to Experience and Understand HF Ionospheric Radio Propagation
Total Page:16
File Type:pdf, Size:1020Kb
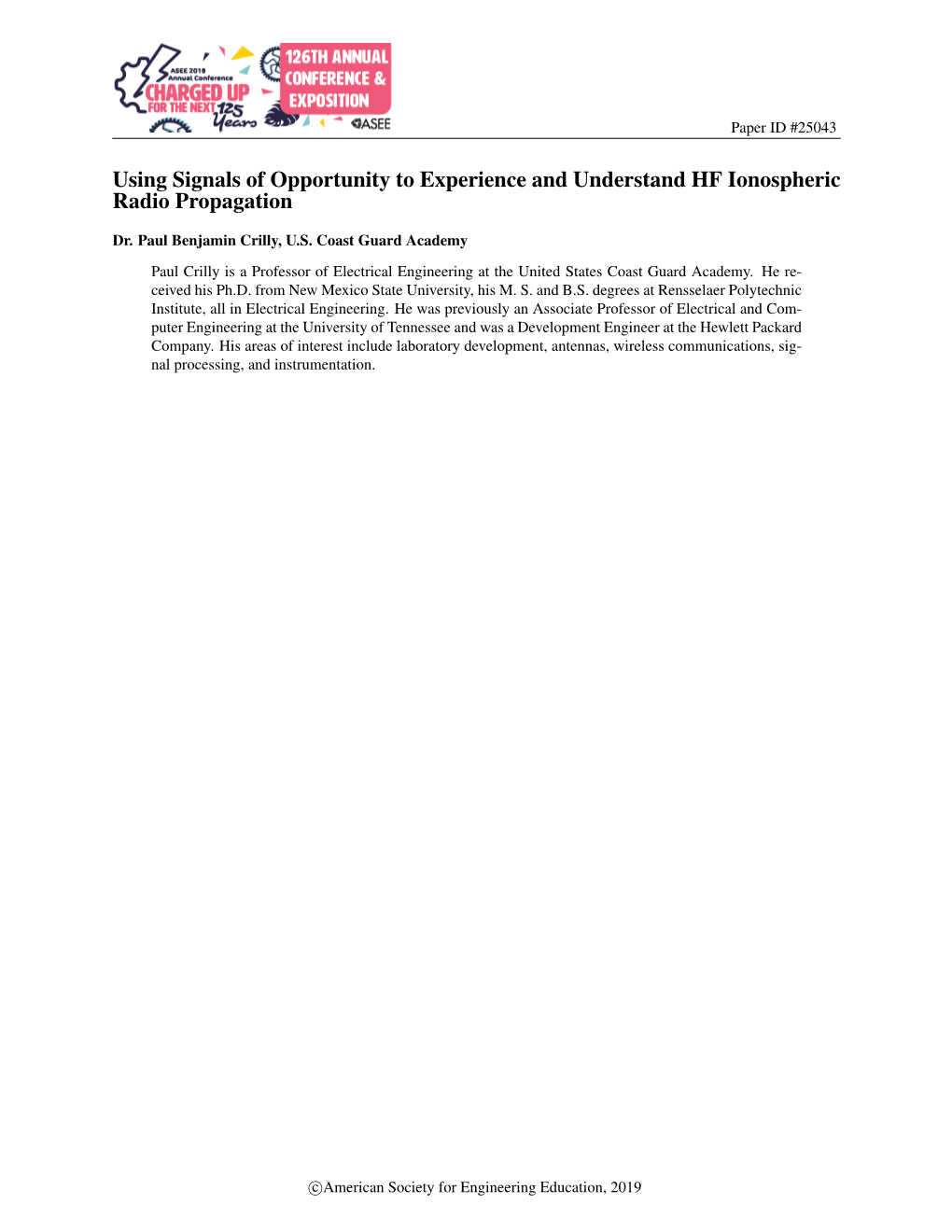
Load more
Recommended publications
-
Atmospheric Anisotrophy and Its Effect on the Delay Power Spectra of Tropospheric Scatter Radio Signals Hosny Mohammed Ibrahim Iowa State University
Iowa State University Capstones, Theses and Retrospective Theses and Dissertations Dissertations 1982 Atmospheric anisotrophy and its effect on the delay power spectra of tropospheric scatter radio signals Hosny Mohammed Ibrahim Iowa State University Follow this and additional works at: https://lib.dr.iastate.edu/rtd Part of the Electrical and Electronics Commons Recommended Citation Ibrahim, Hosny Mohammed, "Atmospheric anisotrophy and its effect on the delay power spectra of tropospheric scatter radio signals " (1982). Retrospective Theses and Dissertations. 7507. https://lib.dr.iastate.edu/rtd/7507 This Dissertation is brought to you for free and open access by the Iowa State University Capstones, Theses and Dissertations at Iowa State University Digital Repository. It has been accepted for inclusion in Retrospective Theses and Dissertations by an authorized administrator of Iowa State University Digital Repository. For more information, please contact [email protected]. INFORMATION TO USERS This reproduction was made from a copy of a document sent to us for microfilming. While the most advanced technology has been used to photograph and reproduce this document, the quality of the reproduction is heavily dependent upon the quality of the material submitted. The following explanation of techniques is provided to help clarify markings or notations which may appear on this reproduction. 1. The sign or "target" for pages apparently lacking from the document photographed is "Missing Page(s)". If it was possible to obtain the missing page(s) or section, they are spliced into the film along with adjacent pages. This may have necessitated cutting through an image and duplicating adjacent pages to assure complete continuity. -
Proposed Changes to the Morse Code (CW) ) RM-10784, Proficiency Requirement for Operator ) RM-10785, Access to the Amateur Radio Bands ) RM-10786, and Below 30 Mhz
Before the Federal Communications Commission Washington, DC 20554 ) In the Matter of ) RM-10781, ) RM-10782, The Amateur Radio Service: ) RM-10783, Proposed Changes to the Morse Code (CW) ) RM-10784, Proficiency Requirement for Operator ) RM-10785, Access to the Amateur Radio Bands ) RM-10786, and Below 30 MHz. ) RM-10787 ) COMMENTS TO PETITIONS FOR RULEMAKING GREETINGS: INTRODUCTION As all parties concerned are no doubt aware, the Morse code telegraphy proficiency requirement for Amateur Radio Service operators has been eliminated from the International Telecommunications Union (ITU) Radio Regulations. This change was effected on 5 July 2003 at the World Radiocommunication Conference 2003 (WRC-03), Geneva, by revising Article 25.5 §3 1 of these regulations. The revised Article 25.5 now gives the administrations of individual member nations, such as the US Federal Communications Commission (FCC or Commission), discretion to “…determine whether or not a person seeking a license to operate an amateur station shall demonstrate the ability to send and receive texts in Morse code signals.” Previously, knowledge of or demonstration of Morse code proficiency had been required by ITU regulation for amateur radio operation on all frequencies below 30 MHz. These 1 Dixon Comments frequencies include all of the amateur High Frequency (HF or shortwave) bands, and the one amateur Medium Frequency (MF or medium-wave) band. Note: For purposes of this document, references henceforth to “HF” or “High Frequency” or “shortwave” shall be deemed to include MF or Medium Frequency or medium-wave, as well. This is in fact colloquial nomenclature among amateur radio operators. The various petitions for rulemaking captioned above seek various degrees of relief from the somewhat burdensome requirement for US-licensed Amateur Radio Service operators (amateurs), presently needed to access the very popular and preferential international High Frequency amateur radio bands. -
Portable Shortwave Receivers
Portable Shortwave Receivers ● Longwave, AM, FM and Shortwave ELITE SATELLIT ● VHF Air Band ● HD Radio Reception ● RDS Display ● Superior Sensitivity and Selectivity ● Dual Conversion Design ● Huge 5.7 Inch Backlit Display ● Drift-free Digital Phase Lock Loop ● Direct Frequency and Band Entry ● Single Sideband Synchronous Detector ● Selectable Bandwidths ● High Dynamic Range ● Dual Programmable Clocks ● Dual Event Programmable Timers ● Stereo Line Level Input ● Stereo Line Level Output ● Earphone Jack ● Separate Bass and Treble Controls ● Adjustable AGC: Fast or Slow ● Telescopic Antenna AM/FM/SW ● Battery (4xD) or Included AC Adapter ● Scan and Search ● 1700 Total Memories (500 alphanumeric) ● Deluxe Carry Bag The Elite Satellit is simply the finest full-sized portable in the world. The Elite Satellit is an elegant confluence of performance, features and capabilities. The look, feel and finish of this radio is superb. The solid, quality feel is second to none. The digitally synthesized, dual conversion shortwave tuner covers all long wave, mediums wave (AM) and shortwave frequencies. HD Radio improves audio fidelity and adds additional programming without a subscription fee. Adjacent frequency interference can be minimized or eliminated with a choice of three bandwidths [7.0, 4.0, 2.5 kHz]. The sideband selectable Synchronous AM Detector further minimizes adjacent frequency interference and reduces fading distortion of AM signals. IF Passband Tuning is yet another advanced feature that functions in AM and SSB modes to reject interference. AGC is selectable at fast or slow. High dynamic range permits the detection of weak signals in the presence of strong signals. All this coupled with great sensitivity will bring in stations from every part of the globe. -
Recommendation ITU-R V.573-4
Rec. ITU-R V.573-5 1 RECOMMENDATION ITU-R V.573-5* Radiocommunication vocabulary (1978-1982-1986-1990-2000-2007) Scope This Recommendation provides the main vocabulary reference, giving synonymous terms in three languages and the associated definitions. It includes terms given in Article 1 of the Radio Regulations (RR) and extends the list to technical terms defined in texts of the ITU-R. The ITU Radiocommunication Assembly, considering a) that Article 1 of the Radio Regulations (RR) contains the definitions of terms for regulatory purposes; b) that the Radiocommunication Study Groups have a need to establish new and amended definitions for technical terms that do not appear in RR Article 1 or that are so defined as to be unsuitable for Radiocommunication Study Group purposes; c) that it would be desirable for some of these terms and definitions established by the Radiocommunication Study Groups to be more widely used within the ITU-R, recommends that the terms listed in RR Article 1 and in Annex 1 below should be used as far as possible with the meaning ascribed to them in the corresponding definition. NOTE 1 – Study Groups are invited, where there is a difficulty in using any of the terms with the meaning given in the corresponding definition, to forward to the Coordination Committee for Vocabulary (CCV) a proposal for revision or alternative application, accompanied by substantiating argument. NOTE 2 – A number of terms in this Recommendation appear also in RR Article 1 with a different definition. These terms are identified by (RR . ., MOD) or (RR . .(MOD)) if the modifications consist only of editorial changes. -
Radio Astronomy
Edition of 2013 HANDBOOK ON RADIO ASTRONOMY International Telecommunication Union Sales and Marketing Division Place des Nations *38650* CH-1211 Geneva 20 Switzerland Fax: +41 22 730 5194 Printed in Switzerland Tel.: +41 22 730 6141 Geneva, 2013 E-mail: [email protected] ISBN: 978-92-61-14481-4 Edition of 2013 Web: www.itu.int/publications Photo credit: ATCA David Smyth HANDBOOK ON RADIO ASTRONOMY Radiocommunication Bureau Handbook on Radio Astronomy Third Edition EDITION OF 2013 RADIOCOMMUNICATION BUREAU Cover photo: Six identical 22-m antennas make up CSIRO's Australia Telescope Compact Array, an earth-rotation synthesis telescope located at the Paul Wild Observatory. Credit: David Smyth. ITU 2013 All rights reserved. No part of this publication may be reproduced, by any means whatsoever, without the prior written permission of ITU. - iii - Introduction to the third edition by the Chairman of ITU-R Working Party 7D (Radio Astronomy) It is an honour and privilege to present the third edition of the Handbook – Radio Astronomy, and I do so with great pleasure. The Handbook is not intended as a source book on radio astronomy, but is concerned principally with those aspects of radio astronomy that are relevant to frequency coordination, that is, the management of radio spectrum usage in order to minimize interference between radiocommunication services. Radio astronomy does not involve the transmission of radiowaves in the frequency bands allocated for its operation, and cannot cause harmful interference to other services. On the other hand, the received cosmic signals are usually extremely weak, and transmissions of other services can interfere with such signals. -
Shortwave-Listening
Listen to global news in the making! Monitor transmissions from Voice of America. Radio Canada, Radio Netherlands. Radio Moscow and many others. Learn how radio waves tra- vel. Get information on popular shortwave bands and licensing for a Itypes of equipment. Radio Listener's Guide by William Barden, Jr. Radio Shack A Division of Tandy Corporation First Edition First Printing-1987 Copyright01987 by William Barden Jr., Inc. Printed in the United States of America. All rights reserved. Reproduction or use, without express permission, of editorial or pictorial content, in any manner, is prohibited. No patent liability is assumed with respect to the use of the information contained herein. Library of Congress Catalog Card Number: XX-XXXXX Radio Listener's Guide T Table of Contents Section I World of the BBC, Radio Moscow, Police Calls, Aircraft Communications, and Hams Chapter 1. Radio—What Is It' Generating Radio Waves—The Radio Spectrum—Radio Equipment and Frequencies—Band Use—How Radio Waves Travel—Radio Licenses and Listening—Subbands and Channels—Radio Equipment Chapter 2. Types of Broadcasting Voice Communication—Code Transmission—Teleprinter Transmission— Facsimile Transmission—Slow-Scan Television—Fast-Scan Television — Repeaters— Portable Phones—Satellite Reception—Transmitting Power Chapter 3. Shortwave Broadcasters Frequency Assignments—The European Long-Wave Band—The AM Broadcast Band—Tropical Broadcasting-49- and 41-Meter Bands-31- and 25-Meter Bands—Above the 25-Meter Band—A Typical Listening Session—Logging Foreign Stations—Foreign Broadcast lnformation—QSL Cards Chapter 4. Other Types of Broadcasting in the Lower Frequencies Transmissions Below the AM Broadcast Band—The AM Broadcast Band— Portable Phones—Marine Transmissions—CW Transmissions—Radio Teleprinter—Single Sideband—Time and Frequency Signals—Weather Maps by Facsimile—Citizen's Band Frequencies—The Russian Woodpecker—Pirate and Clandestine Stations Chapter 5. -
Modem Equipment for the New Generation Compact Troposcatter Stations
5 UDC 621.396 MODEM EQUIPMENT FOR THE NEW GENERATION COMPACT TROPOSCATTER STATIONS Serhii O. Kravchuk, Mikolay M. Kaydenko National Technical University of Ukraine “KPI”, Kyiv, Ukraine Background. Modem equipment of tropospheric communication lines is an important component of modern means of telecommunication. The theoretical and practical aspects of choosing a preferred embodiment of modem equipment, taking into account the aggregate indicators of quality. Objective. Presentation features the construction of modem equipment of the tropospheric stations of new generation that can provide high data transfer rates with guaranteed quality of service in complex stationary and non-stationary noise inherent in tropospheric channels. Methods. This goal is achieved by using new technical and architectural solutions to build a modem equipment, spectrally efficient modulation types and coding algorithms of effective adaptation to changing operating conditions. Feasibility of the proposed approaches to the construction of the modem hardware is fulfilled on a prototype of the equipment based on the HSMC ARRadio Daughter Card debugging modules. Results. The features of constructing of modem equipment of troposcatter stations with high data transfer rate are provided. To reach the limiting parameters of such stations proposed in the application of modem equipment of new technical and architectural solutions, spectrally efficient modulation types (OFDM plus linear modulation) and error-correcting coding, efficient algorithms of adaptation to changing conditions of work, the SDR technology, frame structures of physical layer. The variants of the configuration of modem equipment in relation to the modes of operation of compact troposcatter station. Conclusions. Ways of improving modem performance to improve the efficiency of modern compact troposcatter radiorelay stations. -
Tropospheric Refraction Modeling Using Ray-Tracing and Parabolic Equation
98 P. VALTR, P. PECHAČ, TROPOSPHERIC REFRACTION MODELING USING RAY-TRACING AND PARABOLIC EQUATION Tropospheric Refraction Modeling Using Ray-Tracing and Parabolic Equation Pavel VALTR, Pavel PECHAČ Dept. of Electromagnetic Field, Czech Technical University in Prague, Technická 2, 166 27 Praha 6, Czech Republic [email protected], [email protected] Abstract. Refraction phenomena that occur in the lower proper method and its implementation for a specific appli- atmosphere significantly influence the performance of cation. At the end a method for angle-of-arrival spectra wireless communication systems. This paper provides an calculation is presented for precise multipath propagation overview of corresponding computational methods. Basic simulations. properties of the lower atmosphere are mentioned. Practi- cal guidelines for radiowave propagation modeling in the lower atmosphere using ray-tracing and parabolic equa- 2. Radio Refractive Index tion methods are given. In addition, a calculation of angle- of-arrival spectra is introduced for multipath propagation The troposphere forms the lowest part of the atmo- simulations. sphere from the surface of the earth up to several km. From the propagation point of view, the troposphere is charac- terized by a refractive index, whereas the rate of the change of the refractive index with height is of crucial importance. Keywords The refractive index itself depends on absolute tempera- ture, atmospheric pressure and partial pressure due to water Radiowave propagation, Tropospheric refraction, vapor [1]. The predominant dependence of these quantities Ray-tracing, Parabolic equation. on elevation makes the troposphere a mostly horizontally stratified media. The refractive properties of air can be expressed in terms of the refractive index n or refractivity 1. -
Spectrophotometric and Colorimetric Study of Diseased and Rust
NATIONAL BUREAU OF STANDARDS REPORT U5sa SPECIROPHOTOMETRIC AND CWLCEXMEIRIC STUD! OF DISEASED AND RUST RESISTING CEREAL CROPS By Harry J« Keegan John C* Schleter Wiley A. Hall, Jr., and QLadys M* Haas To U* S. Department of die Air Force Aerial Recocmaissance laboratory Wright Air De'velopment Center Wright-Patterscn Air Force Base, Ohio U. S. DEPARTMENT OF COMMERCE NATIONAL BUREAU OF STANDARDS . V. S. DEPARTMENT OF COMMERCE Sinclair Weeks, Secretary NATIONAL HLREAU OF STANDARDS A. V. Aslin, Director THE NATIONAL BUREAU OF STANDARDS The scope of activities of the National Bureau of Standards at its headquarters in Washington, D. C., and its major field laboratories in Boulder, Colorado, is suggested in the following listing of the di\ isions and sections engaged in technical work. In general, each section carries out specialized research, development, and engineering in the field indicated by its title. A brief description of the activities, and of the resultant reports and publications, appears on the inside back cover of this re[)ort. WASHINGTON, D. C. Electricity and Electronics. Resistance and Reactance. Electron Tubes. Electrical Instru- ments. Magnetic Measurements. Process Technology. Engineering Electronics. Electronic Instrumentation. Elect roeheniis try. Optics and Metrology. Photometry and Colorimetry. Optical Instruments. Photographic Teehnolog\. Length. Engineering Metrology. H eat and Power. Temp<‘ratur<‘ Measurements. ThermodMiamies. Cryogenic Phvsies. Engines and Lubrication. Engine Fuels. Atomic and Radiation Physics. Speetroseop\ . Kadiometrv . Mass Spectrometry. Solid State Phvsies. Eh^etron Ph\si(‘s. Atomic Phvsies. Nuclear Phvsies. Radioaetivitv. X-rays. B(*tatron. Nuch'onic Instruimmlation. Radiological f.quipment. AEC Radiation Instruments. (dicniistrv. Organic (boatings. Surface ClKunistrv . Organic Clnmiistrv . -
Grundig S800
FRONT PANEL DESCRIPTION (See page 12) LCD DISPLAY DESCRIPTION (See page 14) REAR PANEL DESCRIPTION (See Paee 15) 3 SPECIFICATIONS Frequency Range: 100 to 30.000 kHz (0.1 to 30 MHz). Line Audio Outputs: Stereo left and right. 87 to 108 MHz, 118 to 137 MHz. 300 mV. 4.7K Ohms for each output. AM. USB. LSB modes (0.1 to 30 MHz). AM mode only for 118 to 137 MHz. Headphone Jack: 1/8 inch (3.175mm) stereo/mono type. FM mode only for 87 to 108 MHz. DC Power Requirements: Input: 7-10 VDC 0 1 Amp. supplied from Sensitivity - SSB AC ADAPTER, external DC Power (10 dB S+N/N): Less than 0.5 uV, 0.1 to 30 MHz. Supply or 5.7 to 9.0 VDC supplied by (6) internally mounted "D" cell (1.5V) Sensitivity - AM batteries (not supplied). (10 dB S+N/N): Less than 2.0 uV. 0.1 to 30 MHz. (1000 Hz. 30% Mod): Less than 4.0 pV, 118 to 137 MHz. Current requirements (approximate) from Sensitivity - FM 9.0 VDC Supply or (20 dB S/N) (monaural): Less than 4 uV, 87 to 108 MHz. Batteries with 1/4 W average Audio Output: Mode Dependent: Frequency Stability 10 ppm, 0C to 50C 510 mA minimum with lamp off, 830 mA maximum with lamp on. Frequency Accuracy: Better than 100 Hz. c 25' C Operating Temperature: 0C to +50C Selectivity - SSB. AM: 6 kHz c -6 dB, less than 12 kHz 0 -60 dB. 4 kHz c -6 dB. -
Shortwave Radio Listening
Shortwave Radio Listening What You Need To Get Started Jeffrey P. Miller (N2AWA) / March 2014 (Third Edition) Table of Contents About this Guide ............................................................................................................................. 1 What is Shortwave Radio Listening? .............................................................................................. 1 What Do I Need to Get Started? ..................................................................................................... 2 Shortwave Radio ......................................................................................................................... 2 Program Guide ............................................................................................................................ 3 Convert Your Local Time to Coordinated Universal Time (UTC) ............................................. 3 Where are the Shortwave Frequencies? .......................................................................................... 3 The Shortwave Band Plan (2.3 MHz – 30 MHz) ........................................................................ 4 What Can I Hear on Shortwave? ................................................................................................. 5 What are the Best Times to Listen? ............................................................................................ 5 Daytime and Nighttime Listening .............................................................................................. -
RADIOECTRONICS in ALL ITS PHASES for Better Curves
1 "FLYING SPOT" TELEVISION TUBE SFi- TfIfVISInN SlrtlON RADIOECTRONICS IN ALL ITS PHASES For Better Curves Where They Count Most! Accurate taper curves prove correct resistance ments - and adds manufacturing skill and values. When you install a Mallory carbon repeated quality checks that assure you com- control, you know that your customer will get plete customer satisfaction when Mallory the fine, smooth tone gradations that result products are used. from tapers that are mathematically accurate. Mallory offers the most complete line of Mallory uses an exclusive method of applying volume controls- standardized to make them the talcum -fine carbon so that the fields of easy to stock. resistance are perfectly feathered for core rect attenuation. Mallory makes the three replacement parts that The Mallory 1485 Control Deal This attractive metal cabinet contains the are used on the majority 15 Controls and 9 Switches that will take of your jobs: volume care of 90% of your service calls. Its arrange- ment makes inventory control almost auto- controls, capacitors and matic-saves you frequent trips to the distrib- utor's e ter. It contains a rack for your vibrators. Into them, Radio Service Ency- Mallory builds design clopedia. You pay only for the Volume Con- experience that has been trols and Switches; the cabinet is included in acquired by constantly the deal at no extra keeping a step ahead cost to you. Check your Mallory distributor on Mallory controls are carefully tested J taper The esrlutire method of applying of commercial radio - this special offer. carbon Aires a Inure gradual taper curve than is produced by n elertronir develop.