Osteoblast Oncostatin M Signaling in Modeled Spaceflight
Total Page:16
File Type:pdf, Size:1020Kb
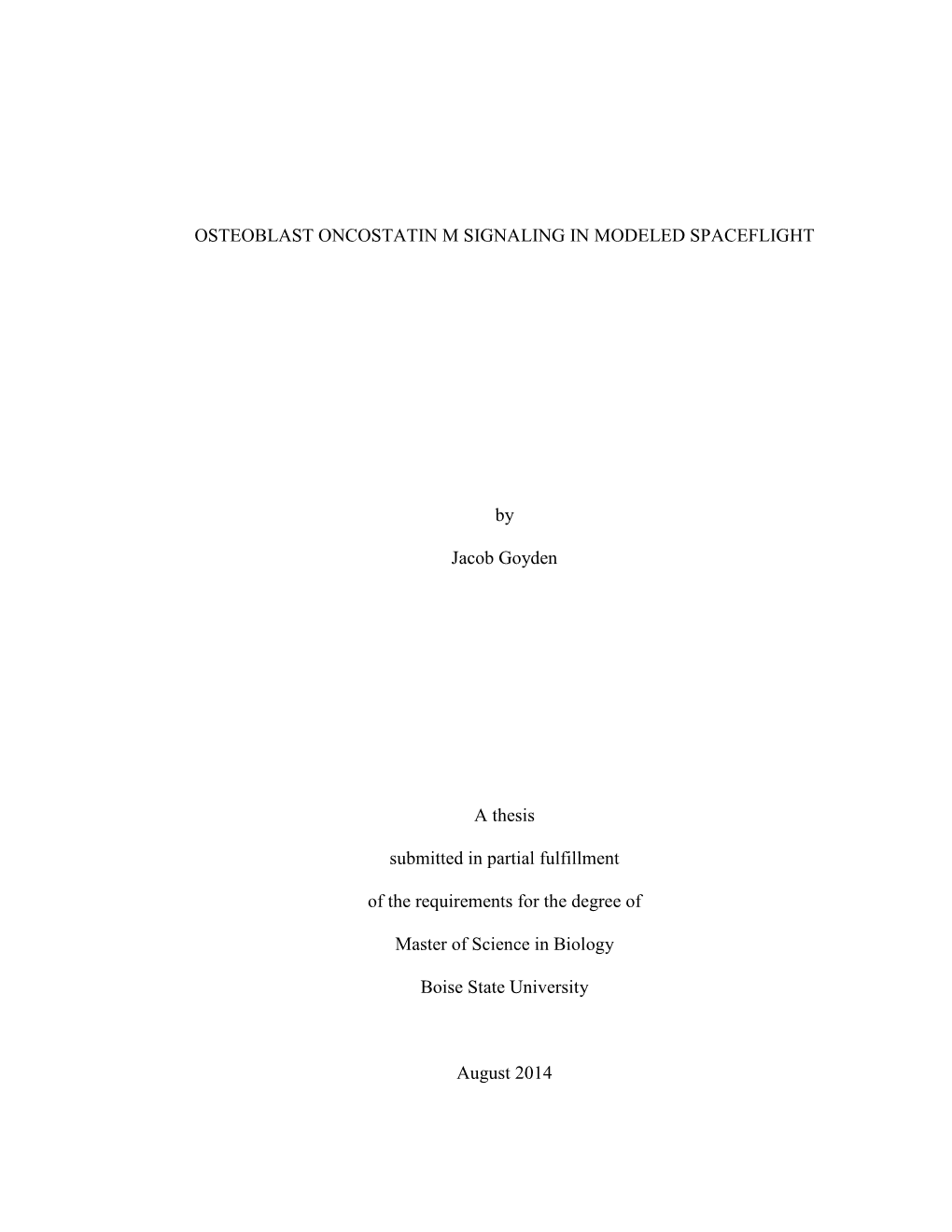
Load more
Recommended publications
-
Supplemental Information to Mammadova-Bach Et Al., “Laminin Α1 Orchestrates VEGFA Functions in the Ecosystem of Colorectal Carcinogenesis”
Supplemental information to Mammadova-Bach et al., “Laminin α1 orchestrates VEGFA functions in the ecosystem of colorectal carcinogenesis” Supplemental material and methods Cloning of the villin-LMα1 vector The plasmid pBS-villin-promoter containing the 3.5 Kb of the murine villin promoter, the first non coding exon, 5.5 kb of the first intron and 15 nucleotides of the second villin exon, was generated by S. Robine (Institut Curie, Paris, France). The EcoRI site in the multi cloning site was destroyed by fill in ligation with T4 polymerase according to the manufacturer`s instructions (New England Biolabs, Ozyme, Saint Quentin en Yvelines, France). Site directed mutagenesis (GeneEditor in vitro Site-Directed Mutagenesis system, Promega, Charbonnières-les-Bains, France) was then used to introduce a BsiWI site before the start codon of the villin coding sequence using the 5’ phosphorylated primer: 5’CCTTCTCCTCTAGGCTCGCGTACGATGACGTCGGACTTGCGG3’. A double strand annealed oligonucleotide, 5’GGCCGGACGCGTGAATTCGTCGACGC3’ and 5’GGCCGCGTCGACGAATTCACGC GTCC3’ containing restriction site for MluI, EcoRI and SalI were inserted in the NotI site (present in the multi cloning site), generating the plasmid pBS-villin-promoter-MES. The SV40 polyA region of the pEGFP plasmid (Clontech, Ozyme, Saint Quentin Yvelines, France) was amplified by PCR using primers 5’GGCGCCTCTAGATCATAATCAGCCATA3’ and 5’GGCGCCCTTAAGATACATTGATGAGTT3’ before subcloning into the pGEMTeasy vector (Promega, Charbonnières-les-Bains, France). After EcoRI digestion, the SV40 polyA fragment was purified with the NucleoSpin Extract II kit (Machery-Nagel, Hoerdt, France) and then subcloned into the EcoRI site of the plasmid pBS-villin-promoter-MES. Site directed mutagenesis was used to introduce a BsiWI site (5’ phosphorylated AGCGCAGGGAGCGGCGGCCGTACGATGCGCGGCAGCGGCACG3’) before the initiation codon and a MluI site (5’ phosphorylated 1 CCCGGGCCTGAGCCCTAAACGCGTGCCAGCCTCTGCCCTTGG3’) after the stop codon in the full length cDNA coding for the mouse LMα1 in the pCIS vector (kindly provided by P. -
Gingival Crevicular Fluid Levels of Sclerostin, Osteoprotegerin, And
Volume 86 • Number 12 Gingival Crevicular Fluid Levels of Sclerostin, Osteoprotegerin, and Receptor Activator of Nuclear Factor-kB Ligand in Periodontitis Umut Balli,* Ahmet Aydogdu,† Figen Ongoz Dede,* Cigdem Coskun Turer,* and Berrak Guven‡ Background: To investigate changes in the levels and rel- ative ratios of sclerostin, osteoprotegerin (OPG), and recep- tor activator of nuclear factor-kB ligand (RANKL) in the gingival crevicular fluid (GCF) of patients with periodontitis after non-surgical periodontal treatment. Methods: Fifty-four individuals (27 healthy controls and 27 patients with chronic periodontitis [CP]) were enrolled in eriodontal disease is a complex the study. Periodontitis patients received non-surgical peri- biologic process related to the in- odontal therapy. GCF sampling and clinical periodontal pa- Pteraction between groups of mi- rameters were assessed before and 6 weeks after therapy. croorganisms and the host immune/ Sclerostin, OPG, and RANKL levels were measured by enzyme- inflammatory response.1 When the bal- linked immunosorbent assay, and their relative ratios were ance between microbial challenge and calculated. host response is disturbed, periodontal Results: Total amounts and concentrations of sclerostin breakdown (clinical attachment loss [AL] were significantly higher in patients with CP than in healthy and alveolar bone resorption) can oc- individuals (P <0.025) and decreased after treatment cur.1,2 Microorganisms and their prod- (P <0.05). The RANKL/OPG ratio was significantly lower in ucts are the primary etiologic factors that healthy individuals than in patients with periodontitis before directly initiate periodontal disease. and after treatment (P <0.025), but no significant difference However, the majority of periodontal was observed in patients with periodontitis after treatment breakdown is caused by endogenous (P >0.05). -
A Model of Inflammatory Arthritis Highlights a Role for Oncostatin M In
Available online http://arthritis-research.com/content/7/1/R57 ResearchVol 7 No 1 article Open Access A model of inflammatory arthritis highlights a role for oncostatin M in pro-inflammatory cytokine-induced bone destruction via RANK/RANKL Wang Hui1, Tim E Cawston1, Carl D Richards2 and Andrew D Rowan1 1Musculoskeletal Research Group, The Medical School, University of Newcastle, Newcastle upon Tyne, UK 2Department of Pathology and Molecular Medicine, McMaster University, Hamilton, Ontario, Canada Corresponding author: Andrew D Rowan, [email protected] Received: 21 Jul 2004 Revisions requested: 20 Sep 2004 Revisions received: 5 Oct 2004 Accepted: 11 Oct 2004 Published: 10 Nov 2004 Arthritis Res Ther 2005, 7:R57-R64 (DOI 10.1186/ar1460)http://arthritis-research.com/content/7/1/R57 © 2004 Hui et al., licensee BioMed Central Ltd. This is an Open Access article distributed under the terms of the Creative Commons Attribution License (http://creativecommons.org/licenses/by/ 2.0), which permits unrestricted use, distribution and reproduction in any medium, provided the original work is cited. Abstract Oncostatin M is a pro-inflammatory cytokine previously shown to RANK and its ligand RANKL in the inflammatory cells, in promote marked cartilage destruction both in vitro and in vivo inflamed synovium and in articular cartilage of knee joints treated when in combination with IL-1 or tumour necrosis factor alpha. with the cytokine combinations compared with expression in However, the in vivo effects of these potent cytokine joints treated with the cytokines alone or the control. This model combinations on bone catabolism are unknown. Using of inflammatory arthritis demonstrates that, in vivo, oncostatin M adenoviral gene transfer, we have overexpressed oncostatin M in combination with either IL-1 or tumour necrosis factor alpha in combination with either IL-1 or tumour necrosis factor alpha represents cytokine combinations that promote bone intra-articularly in the knees of C57BL/6 mice. -
Anti-Sclerostin Antibody Inhibits Internalization of Sclerostin and Sclerostin-Mediated Antagonism of Wnt/ LRP6 Signaling
Anti-Sclerostin Antibody Inhibits Internalization of Sclerostin and Sclerostin-Mediated Antagonism of Wnt/ LRP6 Signaling Maarten van Dinther1, Juan Zhang1, Stella E. Weidauer2, Verena Boschert2, Eva-Maria Muth2, Achim Knappik3, David J. J. de Gorter1, Puck B. van Kasteren1¤, Christian Frisch3, Thomas D. Mueller2, Peter ten Dijke1* 1 Department of Molecular Cell Biology and Centre for Biomedical Genetics, Leiden University Medical Center, Leiden, The Netherlands, 2 Department of Molecular Plant Physiology and Biophysics, Julius-von-Sachs Institut, Wuerzburg, Germany, 3 AbD Serotec, a Bio-Rad company, Puchheim, Germany Abstract Sclerosteosis is a rare high bone mass disease that is caused by inactivating mutations in the SOST gene. Its gene product, Sclerostin, is a key negative regulator of bone formation and might therefore serve as a target for the anabolic treatment of osteoporosis. The exact molecular mechanism by which Sclerostin exerts its antagonistic effects on Wnt signaling in bone forming osteoblasts remains unclear. Here we show that Wnt3a-induced transcriptional responses and induction of alkaline phosphatase activity, an early marker of osteoblast differentiation, require the Wnt co-receptors LRP5 and LRP6. Unlike Dickkopf1 (DKK1), Sclerostin does not inhibit Wnt-3a-induced phosphorylation of LRP5 at serine 1503 or LRP6 at serine 1490. Affinity labeling of cell surface proteins with [125I]Sclerostin identified LRP6 as the main specific Sclerostin receptor in multiple mesenchymal cell lines. When cells were challenged with Sclerostin fused to recombinant green fluorescent protein (GFP) this was internalized, likely via a Clathrin-dependent process, and subsequently degraded in a temperature and proteasome-dependent manner. Ectopic expression of LRP6 greatly enhanced binding and cellular uptake of Sclerostin- GFP, which was reduced by the addition of an excess of non-GFP-fused Sclerostin. -
Oncostatin M Is a Proinflammatory Mediator. in Vivo Effects Correlate with Endothelial Cell Expression of Inflammatory Cytokines and Adhesion Molecules
Oncostatin M is a proinflammatory mediator. In vivo effects correlate with endothelial cell expression of inflammatory cytokines and adhesion molecules. V Modur, … , G A Zimmerman, T M McIntyre J Clin Invest. 1997;100(1):158-168. https://doi.org/10.1172/JCI119508. Research Article Oncostatin M is a member of the IL-6 family of cytokines that is primarily known for its effects on cell growth. Endothelial cells have an abundance of receptors for oncostatin M, and may be its primary target. We determined if oncostatin M induces a key endothelial cell function, initiation of the inflammatory response. We found that subcutaneous injection of oncostatin M in mice caused an acute inflammatory reaction. Oncostatin M in vitro stimulated: (a) polymorphonuclear leukocyte (PMN) transmigration through confluent monolayers of primary human endothelial cells; (b) biphasic PMN adhesion through rapid P-selectin expression, and delayed adhesion mediated by E-selectin synthesis; (c) intercellular adhesion molecule-1 and vascular cell adhesion molecule-1 accumulation; and (d) the expression of PMN activators IL-6, epithelial neutrophil activating peptide-78, growth-related cytokine alpha and growth-related cytokine beta without concomitant IL-8 synthesis. The nature of the response to oncostatin M varied with concentration, suggesting high and low affinity oncostatin M receptors independently stimulated specific responses. Immunohistochemistry showed that macrophage-like cells infiltrating human aortic aneurysms expressed oncostatin M, so it is present during a chronic inflammatory reaction. Therefore, oncostatin M, but not other IL-6 family members, fulfills Koch's postulates as an inflammatory mediator. Since its effects on endothelial cells differ significantly from established mediators like TNFalpha, it may uniquely contribute to the inflammatory cycle. -
Investigation of the Underlying Hub Genes and Molexular Pathogensis in Gastric Cancer by Integrated Bioinformatic Analyses
bioRxiv preprint doi: https://doi.org/10.1101/2020.12.20.423656; this version posted December 22, 2020. The copyright holder for this preprint (which was not certified by peer review) is the author/funder. All rights reserved. No reuse allowed without permission. Investigation of the underlying hub genes and molexular pathogensis in gastric cancer by integrated bioinformatic analyses Basavaraj Vastrad1, Chanabasayya Vastrad*2 1. Department of Biochemistry, Basaveshwar College of Pharmacy, Gadag, Karnataka 582103, India. 2. Biostatistics and Bioinformatics, Chanabasava Nilaya, Bharthinagar, Dharwad 580001, Karanataka, India. * Chanabasayya Vastrad [email protected] Ph: +919480073398 Chanabasava Nilaya, Bharthinagar, Dharwad 580001 , Karanataka, India bioRxiv preprint doi: https://doi.org/10.1101/2020.12.20.423656; this version posted December 22, 2020. The copyright holder for this preprint (which was not certified by peer review) is the author/funder. All rights reserved. No reuse allowed without permission. Abstract The high mortality rate of gastric cancer (GC) is in part due to the absence of initial disclosure of its biomarkers. The recognition of important genes associated in GC is therefore recommended to advance clinical prognosis, diagnosis and and treatment outcomes. The current investigation used the microarray dataset GSE113255 RNA seq data from the Gene Expression Omnibus database to diagnose differentially expressed genes (DEGs). Pathway and gene ontology enrichment analyses were performed, and a proteinprotein interaction network, modules, target genes - miRNA regulatory network and target genes - TF regulatory network were constructed and analyzed. Finally, validation of hub genes was performed. The 1008 DEGs identified consisted of 505 up regulated genes and 503 down regulated genes. -
Oncostatin M Regulation of Inflammatory Responses By
Regulation of Inflammatory Responses by Oncostatin M Philip M. Wallace,1,2* John F. MacMaster,† Katherine A. Rouleau,† T. Joseph Brown,* James K. Loy,† Karen L. Donaldson,3* and Alan F. Wahl3* Oncostatin M (OM) is a pleiotropic cytokine produced late in the activation cycle of T cells and macrophages. In vitro it shares properties with related proteins of the IL-6 family of cytokines; however, its in vivo properties and physiological function are as yet ill defined. We show that administration of OM inhibited bacterial LPS-induced production of TNF-a and lethality in a dose-dependent manner. Consistent with these findings, OM potently suppressed inflammation and tissue destruction in murine models of rheumatoid arthritis and multiple sclerosis. T cell function and Ab production were not impaired by OM treatment. Taken together these data indicate the activities of this cytokine in vivo are antiinflammatory without concordant immunosuppression. The Journal of Immunology, 1999, 162: 5547–5555. he normal development of an inflammatory response must from the inflammatory effector phase back to homeostasis also are be rapidly followed by the engagement of a feedback sys- being evaluated for their clinical potential as drugs. The cytokines T tem to minimize adventitious tissue damage and regulate IL-10 and IL-11 both appear to accelerate this process and their the eventual return to homeostasis. This system involves a multi- administration have proven effective in resolving several animal tude of regulators including cytokines, adhesion molecules, pro- models of chronic inflammatory disease (10). teases, corticosteroids, and subsequent regulators of each of these Oncostatin M (OM)4 is a pleiotropic cytokine that is produced agents. -
Crystal Structure and Functional Dissection of the Cytostatic Cytokine
View metadata, citation and similar papers at core.ac.uk brought to you by CORE provided by Elsevier - Publisher Connector Research Article 863 Crystal structure and functional dissection of the cytostatic cytokine oncostatin M Marc C Deller1,2†#, Keith R Hudson2‡#, Shinji Ikemizu1, Jerónimo Bravo1§, E Yvonne Jones1* and John K Heath2 Background: The cytokine oncostatin M (OSM) inhibits growth of Addresses: 1Cancer Research Campaign Receptor certain tumour-derived cells, induces proliferation in other cell types Structure Group, Division of Structural Biology, The Wellcome Trust Centre for Human Genetics, (e.g. haemangioblasts) and is a mediator of inflammatory responses. Its University of Oxford, Roosevelt Drive, Oxford OX3 mechanism of action is via specific binding to gp130 and either the leukaemia 7BN, UK and 2Cancer Research Campaign Growth inhibitory factor receptor (LIFR) or oncostatin M receptor (OSMR) systems Factors Group, School of Biochemistry, University of at the cell surface to form an active signalling complex. Birmingham, Edgbaston, Birmingham B15 2TT, UK. Present addresses: †Yale University School of Results: We report here the crystal structure of human oncostatin M (hOSM) Medicine, Department of Pharmacology, Sterling along with mutagenesis data which map the receptor-binding epitopes of the Hall of Medicine, PO Box 208066, New Haven, molecule. The structure was determined to a resolution of 2.2 Å and conforms CT 06520-8066, USA, ‡Genesis Research and to the haematopoietin cytokine up-up-down-down four-helix bundle topology. Development, 1 Fox Street, Parnell, Auckland, New Zealand and §Laboratory of Molecular Biology, The site 2 epitope, responsible for gp130 binding, is centred around Gly120 Medical Research Council Centre, Hills Road, which forms a ‘dimple’ on the surface of the molecule located on helices A and Cambridge CB2 2QH, UK. -
Oncostatin M Suppresses Activation of IL-17/Th17 Via SOCS3 Regulation in CD4+ T Cells
Oncostatin M Suppresses Activation of IL-17/Th17 via SOCS3 Regulation in CD4 + T Cells This information is current as Hye-Jin Son, Seung Hoon Lee, Seon-Yeong Lee, of September 28, 2021. Eun-Kyung Kim, Eun-Ji Yang, Jae-Kyung Kim, Hyeon-Beom Seo, Sung-Hwan Park and Mi-La Cho J Immunol published online 16 January 2017 http://www.jimmunol.org/content/early/2017/01/15/jimmun ol.1502314 Downloaded from Why The JI? Submit online. http://www.jimmunol.org/ • Rapid Reviews! 30 days* from submission to initial decision • No Triage! Every submission reviewed by practicing scientists • Fast Publication! 4 weeks from acceptance to publication *average by guest on September 28, 2021 Subscription Information about subscribing to The Journal of Immunology is online at: http://jimmunol.org/subscription Permissions Submit copyright permission requests at: http://www.aai.org/About/Publications/JI/copyright.html Author Choice Freely available online through The Journal of Immunology Author Choice option Email Alerts Receive free email-alerts when new articles cite this article. Sign up at: http://jimmunol.org/alerts Errata An erratum has been published regarding this article. Please see next page or: /content/198/12/4879.full.pdf The Journal of Immunology is published twice each month by The American Association of Immunologists, Inc., 1451 Rockville Pike, Suite 650, Rockville, MD 20852 Copyright © 2017 by The American Association of Immunologists, Inc. All rights reserved. Print ISSN: 0022-1767 Online ISSN: 1550-6606. Published January 16, 2017, doi:10.4049/jimmunol.1502314 The Journal of Immunology Oncostatin M Suppresses Activation of IL-17/Th17 via SOCS3 Regulation in CD4+ T Cells Hye-Jin Son,*,1 Seung Hoon Lee,*,1 Seon-Yeong Lee,*,1 Eun-Kyung Kim,* Eun-Ji Yang,* Jae-Kyung Kim,* Hyeon-Beom Seo,* Sung-Hwan Park,*,2 and Mi-La Cho*,†,2 Oncostatin M (OSM) is a pleiotropic cytokine and a member of the IL-6 family. -
Human Induced Pluripotent Stem Cell–Derived Podocytes Mature Into Vascularized Glomeruli Upon Experimental Transplantation
BASIC RESEARCH www.jasn.org Human Induced Pluripotent Stem Cell–Derived Podocytes Mature into Vascularized Glomeruli upon Experimental Transplantation † Sazia Sharmin,* Atsuhiro Taguchi,* Yusuke Kaku,* Yasuhiro Yoshimura,* Tomoko Ohmori,* ‡ † ‡ Tetsushi Sakuma, Masashi Mukoyama, Takashi Yamamoto, Hidetake Kurihara,§ and | Ryuichi Nishinakamura* *Department of Kidney Development, Institute of Molecular Embryology and Genetics, and †Department of Nephrology, Faculty of Life Sciences, Kumamoto University, Kumamoto, Japan; ‡Department of Mathematical and Life Sciences, Graduate School of Science, Hiroshima University, Hiroshima, Japan; §Division of Anatomy, Juntendo University School of Medicine, Tokyo, Japan; and |Japan Science and Technology Agency, CREST, Kumamoto, Japan ABSTRACT Glomerular podocytes express proteins, such as nephrin, that constitute the slit diaphragm, thereby contributing to the filtration process in the kidney. Glomerular development has been analyzed mainly in mice, whereas analysis of human kidney development has been minimal because of limited access to embryonic kidneys. We previously reported the induction of three-dimensional primordial glomeruli from human induced pluripotent stem (iPS) cells. Here, using transcription activator–like effector nuclease-mediated homologous recombination, we generated human iPS cell lines that express green fluorescent protein (GFP) in the NPHS1 locus, which encodes nephrin, and we show that GFP expression facilitated accurate visualization of nephrin-positive podocyte formation in -
The Role of BMP Signaling in Osteoclast Regulation
Journal of Developmental Biology Review The Role of BMP Signaling in Osteoclast Regulation Brian Heubel * and Anja Nohe * Department of Biological Sciences, University of Delaware, Newark, DE 19716, USA * Correspondence: [email protected] (B.H.); [email protected] (A.N.) Abstract: The osteogenic effects of Bone Morphogenetic Proteins (BMPs) were delineated in 1965 when Urist et al. showed that BMPs could induce ectopic bone formation. In subsequent decades, the effects of BMPs on bone formation and maintenance were established. BMPs induce proliferation in osteoprogenitor cells and increase mineralization activity in osteoblasts. The role of BMPs in bone homeostasis and repair led to the approval of BMP 2 by the Federal Drug Administration (FDA) for anterior lumbar interbody fusion (ALIF) to increase the bone formation in the treated area. However, the use of BMP 2 for treatment of degenerative bone diseases such as osteoporosis is still uncertain as patients treated with BMP 2 results in the stimulation of not only osteoblast mineralization, but also osteoclast absorption, leading to early bone graft subsidence. The increase in absorption activity is the result of direct stimulation of osteoclasts by BMP 2 working synergistically with the RANK signaling pathway. The dual effect of BMPs on bone resorption and mineralization highlights the essential role of BMP-signaling in bone homeostasis, making it a putative therapeutic target for diseases like osteoporosis. Before the BMP pathway can be utilized in the treatment of osteoporosis a better understanding of how BMP-signaling regulates osteoclasts must be established. Keywords: osteoclast; BMP; osteoporosis Citation: Heubel, B.; Nohe, A. The Role of BMP Signaling in Osteoclast Regulation. -
Hippo Signaling Interactions with Wnt/Β-Catenin and Notch Signaling Repress Liver Tumorigenesis
The Journal of Clinical Investigation RESEARCH ARTICLE Hippo signaling interactions with Wnt/β-catenin and Notch signaling repress liver tumorigenesis Wantae Kim,1,2 Sanjoy Kumar Khan,1,2 Jelena Gvozdenovic-Jeremic,1 Youngeun Kim,3 Jason Dahlman,1 Hanjun Kim,1,2 Ogyi Park,4 Tohru Ishitani,5 Eek-hoon Jho,3 Bin Gao,4 and Yingzi Yang1,2 1Genetic Disease Research Branch, National Human Genome Research Institute (NHGRI), NIH, Bethesda, Maryland, USA. 2Department of Developmental Biology, Harvard School of Dental Medicine (HSDM), Boston, Massachusetts, USA. 3Department of Life Sciences, University of Seoul, Seoul, South Korea. 4Section on Liver Biology, National Institute on Alcohol Abuse and Alcoholism (NIAAA), NIH, Bethesda, Maryland, USA. 5Division of Cell Regulation Systems, Medical Institute of Bioregulation, Kyushu University, Fukuoka, Japan. Malignant tumors develop through multiple steps of initiation and progression, and tumor initiation is of singular importance in tumor prevention, diagnosis, and treatment. However, the molecular mechanism whereby a signaling network of interacting pathways restrains proliferation in normal cells and prevents tumor initiation is still poorly understood. Here, we have reported that the Hippo, Wnt/β-catenin, and Notch pathways form an interacting network to maintain liver size and suppress hepatocellular carcinoma (HCC). Ablation of the mammalian Hippo kinases Mst1 and Mst2 in liver led to rapid HCC formation and activated Yes-associated protein/WW domain containing transcription regulator 1 (YAP/TAZ), STAT3, Wnt/β-catenin, and Notch signaling. Previous work has shown that abnormal activation of these downstream pathways can lead to HCC. Rigorous genetic experiments revealed that Notch signaling forms a positive feedback loop with the Hippo signaling effector YAP/TAZ to promote severe hepatomegaly and rapid HCC initiation and progression.