Efforts Toward the Directed Evolution of a Bacterial Molybdenum-Dependent Aldehyde Oxidase for Use in Synthesis
Total Page:16
File Type:pdf, Size:1020Kb
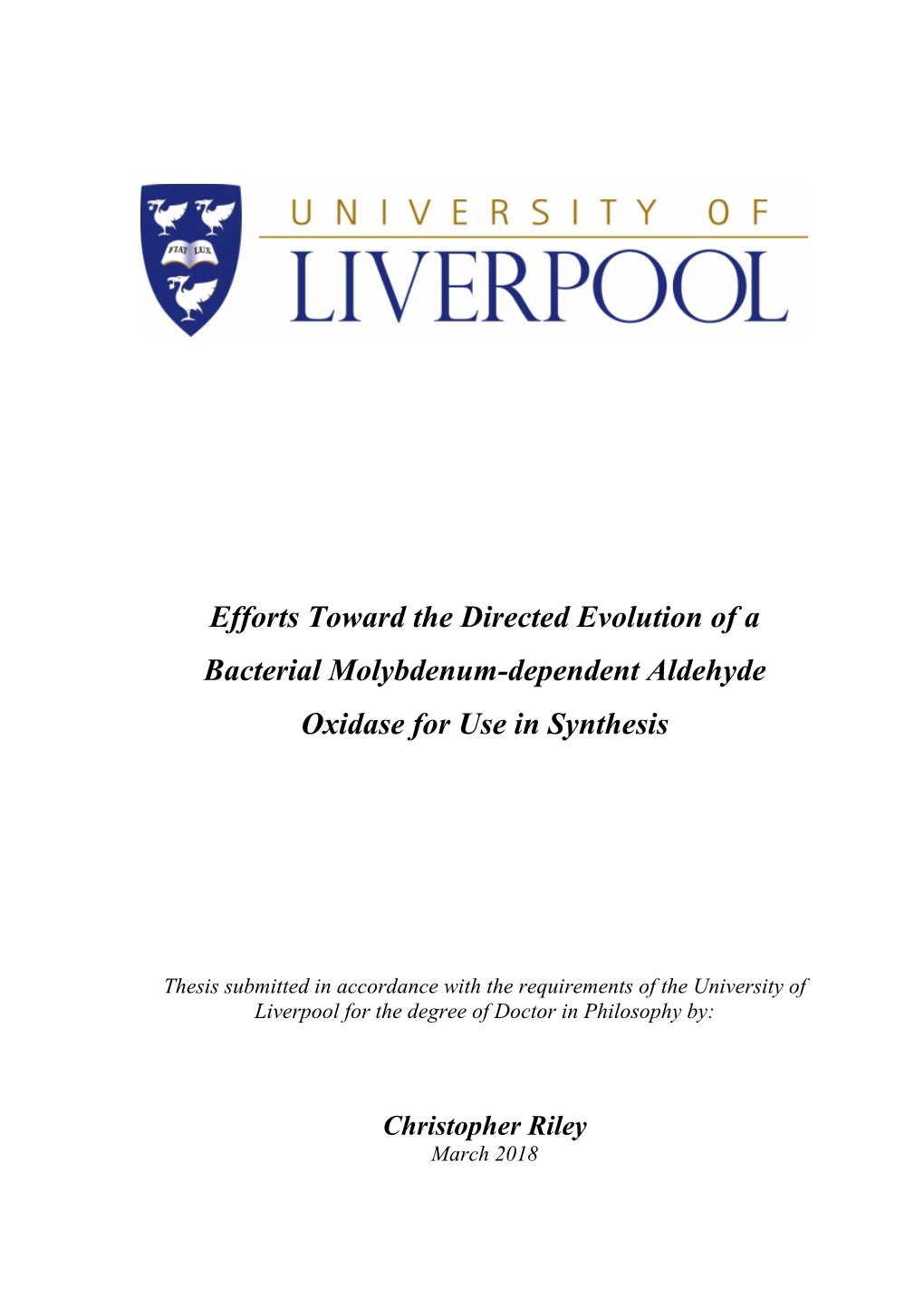
Load more
Recommended publications
-
Corning® Supersomes™ Ultra Human Aldehyde Oxidase
Corning® Supersomes™ Ultra Human Aldehyde Oxidase Aldehyde Oxidase (AO) is a cytosolic enzyme that plays an important role in non-CYP mediated drug metabolism and pharmacokinetics. AO has garnered significant attention in the pharmaceutical industry due to multiple drug failures during clinical trials that were associated with the AO pathway and an increase in the number of aromatic aza-heterocycle moieties found in drug leads that have been identified as substrates for AO. Traditionally, recombinant AO (rAO) is expressed in bacteria. However, this approach has disadvantages such as different protein post-translation modifications that lead to different function as compared to mammalian cells. Corning has developed Corning Supersomes Ultra Aldehyde Oxidase, a recombinant human AO enzyme utilizing a mammalian cell-based expression system to address these issues. This product will enable early assessment of the liability of AO for drug metabolism and clearance. Corning Supersomes Ultra Human Aldehyde Oxidase has been over-expressed in HEK-293 cells and exhibited a significantly higher activity as compared to AO expressed in E. coli. Time- dependent enzyme kinetics, using known substrates and inhibitors, between the rAO and the native form found in human liver cytosol produced a good correlation. Features and Benefits of Corning Supersomes Ultra Aldehyde Oxidase Mammalian cell expression system Corning Supersomes Ultra Human Aldehyde Oxidase Performance Corning Supersomes Ultra AO have been engineered in HEK-293 mammalian cells, thereby eliminating the biosafety concerns Activity Comparison Utilizing Probe Substrate (Zaleplon, 250 µM) associated with baculovirus. Stable and reliable in vitro tool 25 Corning Supersomes Ultra AO are a stable and reliable in vitro tool for the study of AO-mediated metabolism, which provides a 20 quantitative contribution of drug clearance. -
Cytochrome P450 Oxidative Metabolism: Contributions to the Pharmacokinetics, Safety, and Efficacy of Xenobiotics
1521-009X/44/8/1229–1245$25.00 http://dx.doi.org/10.1124/dmd.116.071753 DRUG METABOLISM AND DISPOSITION Drug Metab Dispos 44:1229–1245, August 2016 Copyright ª 2016 by The American Society for Pharmacology and Experimental Therapeutics Special Section on Emerging Novel Enzyme Pathways in Drug Metabolism—Commentary Cytochrome P450 and Non–Cytochrome P450 Oxidative Metabolism: Contributions to the Pharmacokinetics, Safety, and Efficacy of Xenobiotics Robert S. Foti and Deepak K. Dalvie Pharmacokinetics and Drug Metabolism, Amgen, Cambridge, Massachusetts (R.S.F.); and Pharmacokinetics, Dynamics, and Metabolism, Pfizer, La Jolla, California (D.K.D.) Downloaded from Received May 24, 2016; accepted June 10, 2016 ABSTRACT The drug-metabolizing enzymes that contribute to the metabolism this end, this Special Section on Emerging Novel Enzyme Pathways or bioactivation of a drug play a crucial role in defining the in Drug Metabolism will highlight a number of advancements that dmd.aspetjournals.org absorption, distribution, metabolism, and excretion properties of have recently been reported. The included articles support the that drug. Although the overall effect of the cytochrome P450 (P450) important role of non-P450 enzymes in the clearance pathways of family of drug-metabolizing enzymes in this capacity cannot be U.S. Food and Drug Administration–approved drugs over the past understated, advancements in the field of non-P450–mediated me- 10 years. Specific examples will detail recent reports of aldehyde tabolism have garnered increasing attention in recent years. This is oxidase, flavin-containing monooxygenase, and other non-P450 perhaps a direct result of our ability to systematically avoid P450 pathways that contribute to the metabolic, pharmacokinetic, or liabilities by introducing chemical moieties that are not susceptible pharmacodynamic properties of xenobiotic compounds. -
Amino Acid Disorders
471 Review Article on Inborn Errors of Metabolism Page 1 of 10 Amino acid disorders Ermal Aliu1, Shibani Kanungo2, Georgianne L. Arnold1 1Children’s Hospital of Pittsburgh, University of Pittsburgh School of Medicine, Pittsburgh, PA, USA; 2Western Michigan University Homer Stryker MD School of Medicine, Kalamazoo, MI, USA Contributions: (I) Conception and design: S Kanungo, GL Arnold; (II) Administrative support: S Kanungo; (III) Provision of study materials or patients: None; (IV) Collection and assembly of data: E Aliu, GL Arnold; (V) Data analysis and interpretation: None; (VI) Manuscript writing: All authors; (VII) Final approval of manuscript: All authors. Correspondence to: Georgianne L. Arnold, MD. UPMC Children’s Hospital of Pittsburgh, 4401 Penn Avenue, Suite 1200, Pittsburgh, PA 15224, USA. Email: [email protected]. Abstract: Amino acids serve as key building blocks and as an energy source for cell repair, survival, regeneration and growth. Each amino acid has an amino group, a carboxylic acid, and a unique carbon structure. Human utilize 21 different amino acids; most of these can be synthesized endogenously, but 9 are “essential” in that they must be ingested in the diet. In addition to their role as building blocks of protein, amino acids are key energy source (ketogenic, glucogenic or both), are building blocks of Kreb’s (aka TCA) cycle intermediates and other metabolites, and recycled as needed. A metabolic defect in the metabolism of tyrosine (homogentisic acid oxidase deficiency) historically defined Archibald Garrod as key architect in linking biochemistry, genetics and medicine and creation of the term ‘Inborn Error of Metabolism’ (IEM). The key concept of a single gene defect leading to a single enzyme dysfunction, leading to “intoxication” with a precursor in the metabolic pathway was vital to linking genetics and metabolic disorders and developing screening and treatment approaches as described in other chapters in this issue. -
Supplementary Materials
Supplementary Materials COMPARATIVE ANALYSIS OF THE TRANSCRIPTOME, PROTEOME AND miRNA PROFILE OF KUPFFER CELLS AND MONOCYTES Andrey Elchaninov1,3*, Anastasiya Lokhonina1,3, Maria Nikitina2, Polina Vishnyakova1,3, Andrey Makarov1, Irina Arutyunyan1, Anastasiya Poltavets1, Evgeniya Kananykhina2, Sergey Kovalchuk4, Evgeny Karpulevich5,6, Galina Bolshakova2, Gennady Sukhikh1, Timur Fatkhudinov2,3 1 Laboratory of Regenerative Medicine, National Medical Research Center for Obstetrics, Gynecology and Perinatology Named after Academician V.I. Kulakov of Ministry of Healthcare of Russian Federation, Moscow, Russia 2 Laboratory of Growth and Development, Scientific Research Institute of Human Morphology, Moscow, Russia 3 Histology Department, Medical Institute, Peoples' Friendship University of Russia, Moscow, Russia 4 Laboratory of Bioinformatic methods for Combinatorial Chemistry and Biology, Shemyakin-Ovchinnikov Institute of Bioorganic Chemistry of the Russian Academy of Sciences, Moscow, Russia 5 Information Systems Department, Ivannikov Institute for System Programming of the Russian Academy of Sciences, Moscow, Russia 6 Genome Engineering Laboratory, Moscow Institute of Physics and Technology, Dolgoprudny, Moscow Region, Russia Figure S1. Flow cytometry analysis of unsorted blood sample. Representative forward, side scattering and histogram are shown. The proportions of negative cells were determined in relation to the isotype controls. The percentages of positive cells are indicated. The blue curve corresponds to the isotype control. Figure S2. Flow cytometry analysis of unsorted liver stromal cells. Representative forward, side scattering and histogram are shown. The proportions of negative cells were determined in relation to the isotype controls. The percentages of positive cells are indicated. The blue curve corresponds to the isotype control. Figure S3. MiRNAs expression analysis in monocytes and Kupffer cells. Full-length of heatmaps are presented. -
Supplementary Table S4. FGA Co-Expressed Gene List in LUAD
Supplementary Table S4. FGA co-expressed gene list in LUAD tumors Symbol R Locus Description FGG 0.919 4q28 fibrinogen gamma chain FGL1 0.635 8p22 fibrinogen-like 1 SLC7A2 0.536 8p22 solute carrier family 7 (cationic amino acid transporter, y+ system), member 2 DUSP4 0.521 8p12-p11 dual specificity phosphatase 4 HAL 0.51 12q22-q24.1histidine ammonia-lyase PDE4D 0.499 5q12 phosphodiesterase 4D, cAMP-specific FURIN 0.497 15q26.1 furin (paired basic amino acid cleaving enzyme) CPS1 0.49 2q35 carbamoyl-phosphate synthase 1, mitochondrial TESC 0.478 12q24.22 tescalcin INHA 0.465 2q35 inhibin, alpha S100P 0.461 4p16 S100 calcium binding protein P VPS37A 0.447 8p22 vacuolar protein sorting 37 homolog A (S. cerevisiae) SLC16A14 0.447 2q36.3 solute carrier family 16, member 14 PPARGC1A 0.443 4p15.1 peroxisome proliferator-activated receptor gamma, coactivator 1 alpha SIK1 0.435 21q22.3 salt-inducible kinase 1 IRS2 0.434 13q34 insulin receptor substrate 2 RND1 0.433 12q12 Rho family GTPase 1 HGD 0.433 3q13.33 homogentisate 1,2-dioxygenase PTP4A1 0.432 6q12 protein tyrosine phosphatase type IVA, member 1 C8orf4 0.428 8p11.2 chromosome 8 open reading frame 4 DDC 0.427 7p12.2 dopa decarboxylase (aromatic L-amino acid decarboxylase) TACC2 0.427 10q26 transforming, acidic coiled-coil containing protein 2 MUC13 0.422 3q21.2 mucin 13, cell surface associated C5 0.412 9q33-q34 complement component 5 NR4A2 0.412 2q22-q23 nuclear receptor subfamily 4, group A, member 2 EYS 0.411 6q12 eyes shut homolog (Drosophila) GPX2 0.406 14q24.1 glutathione peroxidase -
Conversion of Indole-3-Acetaldehyde to Indole-3-Acetic Acid in Cell-Wall Fraction of Barley {Hordeum Vulgare) Seedlings
Plant Cell Physiol. 38(3): 268-273 (1997) JSPP © 1997 Conversion of Indole-3-Acetaldehyde to Indole-3-Acetic Acid in Cell-Wall Fraction of Barley {Hordeum vulgare) Seedlings Ken-ichi Tsurusaki1, Kazuyoshi Takeda2 and Naoki Sakurai3 1 Faculty of Liberal Arts, Fukuyama University, Fukuyama, 729-02 Japan 2 Research Institute for Bioresources, Okayama University, Kurashiki, Okayama, 710 Japan 3 Department of Environmental Studies, Faculty of Integrated Arts & Sciences, Hiroshima University, Higashi-Hiroshima, 739 Japan The cell-wall fraction of barley seedlings was able (Trp) has been suggested as a primary precursor of IAA to oxidize indole-3-acetaldehyde (IAAld) to form IAA, (Gordon 1954, Gibson et al. 1972, Monteiro et al. 1988, whereas the fraction did not catalyze the conversion of in- Cooney and Nonhebel 1991, Bialek et al. 1992, Koshiba dole-3-acetonitrile or indole-3-acetamide to IAA. The activ- and Matsuyama 1993, Koshiba et al. 1995), because Trp iDownloaded from https://academic.oup.com/pcp/article/38/3/268/1928462 by guest on 24 September 2021 s ity was lower in a semi-dwarf mutant that had an endog- similar in structure to IAA and is ubiquitous in plant enous IAA level lower than that of the normal isogenic tissues. strain [Inouhe et al. (1982) Plant Cell Physiol. 23: 689]. Two pathways of IAA biosynthesis from L-Trp have The soluble fraction also contained some activity; the activ- been proposed in higher plants: Trp —• indole-3-pyruvic ity was similar in the normal and mutant strains. The op- acid -»indole-3-acetaldehyde (IAAld) ->• IAA; or Trp -> timal pH for the conversion of IAAld to IAA in the cell- tryptamine —• IAAld -* IAA. -
Index of Recommended Enzyme Names
Index of Recommended Enzyme Names EC-No. Recommended Name Page 1.2.1.10 acetaldehyde dehydrogenase (acetylating) 115 1.2.1.38 N-acetyl-y-glutamyl-phosphate reductase 289 1.2.1.3 aldehyde dehydrogenase (NAD+) 32 1.2.1.4 aldehyde dehydrogenase (NADP+) 63 1.2.99.3 aldehyde dehydrogenase (pyrroloquinoline-quinone) 578 1.2.1.5 aldehyde dehydrogenase [NAD(P)+] 72 1.2.3.1 aldehyde oxidase 425 1.2.1.31 L-aminoadipate-semialdehyde dehydrogenase 262 1.2.1.19 aminobutyraldehyde dehydrogenase 195 1.2.1.32 aminomuconate-semialdehyde dehydrogenase 271 1.2.1.29 aryl-aldehyde dehydrogenase 255 1.2.1.30 aryl-aldehyde dehydrogenase (NADP+) 257 1.2.3.9 aryl-aldehyde oxidase 471 1.2.1.11 aspartate-semialdehyde dehydrogenase 125 1.2.1.6 benzaldehyde dehydrogenase (deleted) 88 1.2.1.28 benzaldehyde dehydrogenase (NAD+) 246 1.2.1.7 benzaldehyde dehydrogenase (NADP+) 89 1.2.1.8 betaine-aldehyde dehydrogenase 94 1.2.1.57 butanal dehydrogenase 372 1.2.99.2 carbon-monoxide dehydrogenase 564 1.2.3.10 carbon-monoxide oxidase 475 1.2.2.4 carbon-monoxide oxygenase (cytochrome b-561) 422 1.2.1.45 4-carboxy-2-hydroxymuconate-6-semialdehyde dehydrogenase .... 323 1.2.99.6 carboxylate reductase 598 1.2.1.60 5-carboxymethyl-2-hydroxymuconic-semialdehyde dehydrogenase . 383 1.2.1.44 cinnamoyl-CoA reductase 316 1.2.1.68 coniferyl-aldehyde dehydrogenase 405 1.2.1.33 (R)-dehydropantoate dehydrogenase 278 1.2.1.26 2,5-dioxovalerate dehydrogenase 239 1.2.1.69 fluoroacetaldehyde dehydrogenase 408 1.2.1.46 formaldehyde dehydrogenase 328 1.2.1.1 formaldehyde dehydrogenase (glutathione) -
Developmental Changes of Aldehyde Oxidase Activity and Protein Expression in Human Liver Cytosol
Drug Metab. Pharmacokinet. 27 (5): 543547 (2012). Copyright © 2012 by the Japanese Society for the Study of Xenobiotics (JSSX) Note Developmental Changes of Aldehyde Oxidase Activity and Protein Expression in Human Liver Cytosol Yoshitaka TAYAMA1,*,KazumiSUGIHARA1,2,SeigoSANOH2, Katsushi MIYAKE1, Shigeyuki KITAMURA2,3 and Shigeru OHTA2 1Faculty of Pharmaceutical Science, Hiroshima International University, Kure, Japan 2Division of Medicinal Chemistry, Graduate School of Biomedical Sciences, Hiroshima University, Hiroshima, Japan 3Nihon Pharmaceutical University, Saitama, Japan Full text of this paper is available at http://www.jstage.jst.go.jp/browse/dmpk Summary: Aldehyde oxidase (AO) plays a role in metabolizing many drugs, such as methotrexate and 6- mercaptopurine. We previously showed that AO activity in rat liver rapidly increases from birth, reaching a plateau within 4 weeks, and is regulated at the protein expression level. However, developmental changes of AO activity and protein expression in human liver have not been reported. Here, we investigated the developmental changes and variability of AO in 16 human livers (13 children ranging from 13 days to 12 years old and 3 adults, 17, 34 and 45 years old). Young children (13 days to 4 months after birth) showed little liver AO activity, evaluated in terms of the activities for oxidation of N-1-methylnicotinamide to N-1- methyl-2-pyridone-5-carboxamide and N-1-methyl-4-pyridone-3-carboxamide in liver cytosol. However, these oxidase activities were markedly increased after 4 months, reaching the adult level by about 2 years of age. The AO band density in immunoblotting analysis waswellcorrelatedwiththeAOactivityamongall subjects (p < 0.01, r2 = 0.771). -
Functional Studies on Oligotropha Carboxidovorans Molybdenum
Functional studies on Oligotropha carboxidovorans molybdenum-copper CO dehydrogenase produced in Escherichia coli Paul Kaufmann1, Benjamin R. Duffus1, Christian Teutloff2 and Silke Leimkühler1* From the 1Institute of Biochemistry and Biology, Department of Molecular Enzymology, University of Potsdam, 14476 Potsdam, Germany. 2Institute for Experimental Physics, Free University of Berlin, Arnimallee 14, 14195 Berlin, Germany. *corresponding author: Silke Leimkühler; Department of Molecular Enzymology, Institute of Biochemistry and Biology, University of Potsdam, Karl-Liebknecht-Str. 24-25, 14476 Potsdam, Germany; Tel.: +49-331-977-5603; Fax: +49-331-977-5128; E-mail: sleim@uni- potsdam.de Running title: Studies on a molybdenum-copper CO dehydrogenase expressed in E. coli 1 The abbreviations used are: molybdenum cofactor (Moco), molybdopterin (MPT), bis-MPT guanine dinucleotide (bis-MGD), carbon monoxide ehydrogenase (CODH), cytidine-5’-monophosphate (5'CMP), high-performance liquid chromatography (HPLC), electron paramagnetic resonance (EPR), ethylenediaminetetraacetic acid (EDTA), g-factor (g). 2 ABSTRACT The Mo/Cu-dependent CO dehydrogenase (CODH) from Oligotropha carboxidovorans is an enzyme that is able to catalyze both the oxidation of CO to CO2 and the oxidation of H2 to protons and electrons. Despite the close to atomic resolution structure (1.1 Å), significant uncertainties have remained with regard to the reaction mechanism of substrate oxidation at the unique Mo/Cu-center, as well as the nature of intermediates formed during the catalytic cycle. So far the investigation of the role of amino acids at the active site was hampered due to the lack of a suitable expression system that allowed for detailed site-directed mutagenesis studies at the active-site. -
Iron Deficiency in the Rat: Biochemical Studies of Brain Metabolism
Pediat. Res. 12: 217-220 (1978) Aldehyde oxidase brain metabolism iron deficiency Iron Deficiency in the Rat: Biochemical Studies of Brain Metabolism BRUCE MACKLER,'24' RICHARD PERSON, LOUISE R. MILLER, A. R. INAMDAR, AND C. A. FINCH Departments of Pediatrics and Medicine, and Center for Child Development and Mental Retardation, University of Washington, Seattle, Washington, USA Summary Mitochondria were prepared from liver as described previ- ously (13). ~itochondiiawere prepared from brain as follows. Studies were performed to determine the effects of iron After removal from the calvarium the brains (4-5 g total) were deficiency on brain metabolism in rats. Concentrations of cyto- placed in 20 ml of a'solution (0-5") of 0.22 M mannitol, 0,08 M chrome pigments, oxidative phosphorylation, and catalase and sucrose, 0.2 mM EDTA, and 5 mM Tris, pH 7.4 (MSET monoamine oxidase activities in brain tissue were unaffected by solution).. All subsequent procedures were carried out at 0-5". iron deficiency. However, activities of aldehyde oxidase, a key The brains were rapidly minced with a sharp scissors, rinsed one enzyme in the pathway of serotonin degradation, were signifi- time with an additional 20 ml cold MSET solution, and sus- cantlv reduced. and concentrations of serotonin and total 5- pended in 6 ml MSET solution/g tissue. The' suspension was hydroxyindole compounds were elevated in brain tissue of iron- gently homogenized at low speed in a motor-driven Teflon-glass deficient animals. Aldehyde oxidase activities and concentra- homogenizer and 0.5 ml of a solution containing 1 mg Nagase, tions of 5-hydroxyindole compounds in brain tissues returned to 1 mg bovine serum albumin (fraction V), and 5 mg KHCO,/ml approximately normal values one week after treatment of iron was added16 ml suspension. -
Nimesulide Increases the Aldehyde Oxidase Activity of Humans and Rats
www.nature.com/aps ARTICLE Nimesulide increases the aldehyde oxidase activity of humans and rats Lei Zhou1, Xiao-yan Pang1, Xiang-yu Hou1, Lu Liu1, Zi-tao Guo1 and Xiao-yan Chen1 An increasing number of drugs are metabolized by aldehyde oxidase (AOX), but AOX-mediated drug interactions are seldom reported due to the lack of appropriate inhibitors and inducers. A recent study reported that nimesulide (NIM) could increase the liver injury risk of methotrexate. The latter was mainly metabolized by AOX to form hepatotoxic 7-hydroxymethotrexate (7-OH MTX). Thus, we speculated that NIM could induce AOX. In this study, we investigated the potential induction of AOX activity by NIM using methotrexate as the probe substrate. Treatment of primary human and rat hepatocytes with NIM (20 μM) for 24 h caused a 2.0- and 3.1-fold, respectively, increase in 7-OH MTX formation. Oral administration of NIM (100 mg·kg−1·d−1, for 5 days) to rats significantly increased the systematic exposure (6.5-fold), liver distribution (2.5-fold), and excretion (5.2-fold for urinary excretion and 2.1-fold for fecal excretion) of 7-OH MTX. The 7-OH MTX formation in liver cytosol from rats pretreated with 20, 50, and 100 mg·kg−1·d−1 NIM for 5 days increased by 1.9-, 3.2-, and 3.7-fold, respectively, compared with that of rats pretreated with the vehicle. We revealed that the elevation of AOX activity was accompanied by an increase in AOX1 protein levels but not the corresponding mRNA levels. -
Supplemental Figures 04 12 2017
Jung et al. 1 SUPPLEMENTAL FIGURES 2 3 Supplemental Figure 1. Clinical relevance of natural product methyltransferases (NPMTs) in brain disorders. (A) 4 Table summarizing characteristics of 11 NPMTs using data derived from the TCGA GBM and Rembrandt datasets for 5 relative expression levels and survival. In addition, published studies of the 11 NPMTs are summarized. (B) The 1 Jung et al. 6 expression levels of 10 NPMTs in glioblastoma versus non‐tumor brain are displayed in a heatmap, ranked by 7 significance and expression levels. *, p<0.05; **, p<0.01; ***, p<0.001. 8 2 Jung et al. 9 10 Supplemental Figure 2. Anatomical distribution of methyltransferase and metabolic signatures within 11 glioblastomas. The Ivy GAP dataset was downloaded and interrogated by histological structure for NNMT, NAMPT, 12 DNMT mRNA expression and selected gene expression signatures. The results are displayed on a heatmap. The 13 sample size of each histological region as indicated on the figure. 14 3 Jung et al. 15 16 Supplemental Figure 3. Altered expression of nicotinamide and nicotinate metabolism‐related enzymes in 17 glioblastoma. (A) Heatmap (fold change of expression) of whole 25 enzymes in the KEGG nicotinate and 18 nicotinamide metabolism gene set were analyzed in indicated glioblastoma expression datasets with Oncomine. 4 Jung et al. 19 Color bar intensity indicates percentile of fold change in glioblastoma relative to normal brain. (B) Nicotinamide and 20 nicotinate and methionine salvage pathways are displayed with the relative expression levels in glioblastoma 21 specimens in the TCGA GBM dataset indicated. 22 5 Jung et al. 23 24 Supplementary Figure 4.