The Synthesis of Peroxyesters
Total Page:16
File Type:pdf, Size:1020Kb
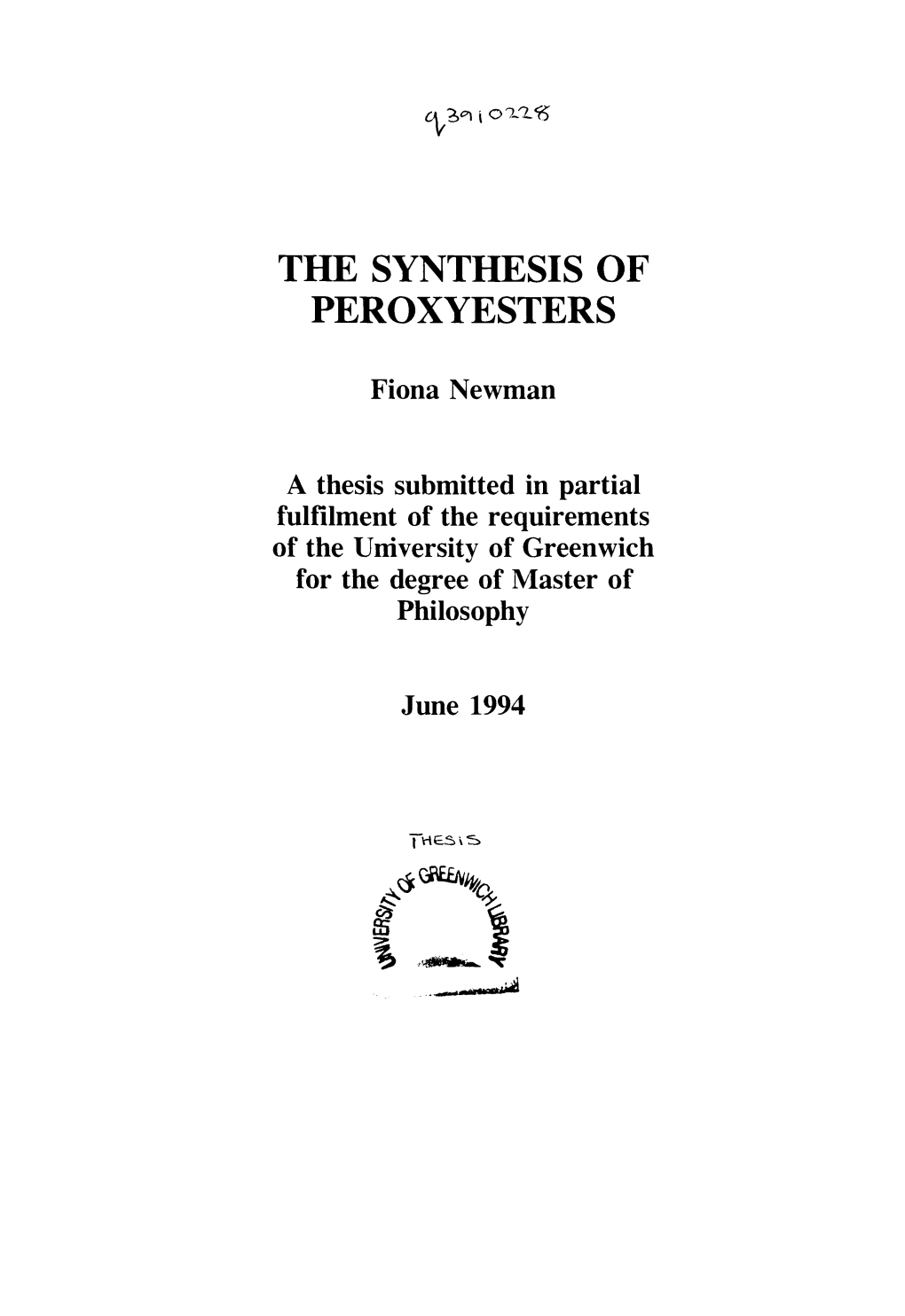
Load more
Recommended publications
-
Cinnamoyl Esters of Lesquerella and Castor Oil: Novel Sunscreen Active Ingredients David L
Cinnamoyl Esters of Lesquerella and Castor Oil: Novel Sunscreen Active Ingredients David L. Compton*, Joseph A. Laszlo, and Terry A. Isbell New Crops and Processing Technology Research Unit, USDA, ARS, NCAUR, Peoria, Illinois 61604 ABSTRACT: Lesquerella and castor oils were esterified with querolin (2). Therefore, LO in essence contains two moles of cinnamic acid (CA) and 4-methoxycinnamic acid (MCA). Esteri- hydroxy functionality per mole of triglyceride (TG). fication of the hydroxy oils reached 85% completion with CA The hydroxy functionality of the FA of the TG can be ex- and 50% conversion with MCA. The hydroxy oils were esteri- ploited by esterification to form estolides. The majority of re- fied at 200°C under a nitrogen atmosphere within a sealed sys- search has focused on the estolides of hydroxy FA and TG tem. Unreacted CA and MCA were removed from the reaction formed with oleic acid. The esterification of hydroxy TG and mixtures by sublimation at 100°C under vacuum. The resultant free lesquerolic acid with oleic acid using a cobalt catalyst (4) methoxycinnamic oils possessed a broader, more blue-shifted or a lipase catalyst (5) has been reported. Also, the acid-cat- UV absorbance, 250 to 345 nm with a λmax of 305 nm, com- pared with the cinnamic oils, which absorbed from 260 to 315 alyzed formation of estolides from CO and LO with oleic acid has been patented (6). Recently, a detailed study of the effect nm, λmax of 270 nm. The methoxycinnamic oils provide better UV-B absorption and thus are better candidates to be used as of oleic acid concentration and temperature on catalyst-free sunscreen active ingredients. -
Unit One Part 2: Naming and Functional Groups
gjr-–- 1 Unit One Part 2: naming and functional groups • To write and interpret IUPAC names for small, simple molecules • Identify some common functional groups found in organic molecules O CH3 H3C N H N O N N O H3C S N O N H3C viagra™ (trade name) sildenafil (trivial name) 5-(2-ethoxy-5-(4-methylpiperazin-1-ylsulfonyl)phenyl)-1-methyl-3-propyl-1H- pyrazolo[4,3-d] pyrimidin-7(6H)-one dr gareth rowlands; [email protected]; science tower a4.12 http://www.massey.ac.nz/~gjrowlan gjr-–- 2 Systematic (IUPAC) naming PREFIX PARENT SUFFIX substituents / minor number of C principal functional functional groups AND multiple bond group index • Comprises of three main parts • Note: multiple bond index is always incorporated in parent section No. Carbons Root No. Carbons Root Multiple-bond 1 meth 6 hex Bond index 2 eth 7 hept C–C an(e) 3 prop 8 oct C=C en(e) 4 but 9 non C≡C yn(e) 5 pent 10 dec gjr-–- 3 Systematic (IUPAC) naming: functional groups Functional group Structure Suffix Prefix General form O –oic acid acid –carboxylic acid carboxy R-COOH R OH O O –oic anhydride anhydride –carboxylic anhydride R-C(O)OC(O)-R R O R O –oyl chloride acyl chloride -carbonyl chloride chlorocarbonyl R-COCl R Cl O –oate ester –carboxylate alkoxycarbonyl R-COOR R OR O –amide amide –carboxamide carbamoyl R-CONH2 R NH2 nitrile R N –nitrile cyano R-C≡N O –al aldehyde –carbaldehyde oxo R-CHO R H O ketone –one oxo R-CO-R R R alcohol R OH –ol hydroxy R-OH amine R NH2 –amine amino R-NH2 O ether R R –ether alkoxy R-O-R alkyl bromide bromo R-Br (alkyl halide) R Br (halo) (R-X) gjr-–- 4 Nomenclature rules 1. -
Polymer Exemption Guidance Manual POLYMER EXEMPTION GUIDANCE MANUAL
United States Office of Pollution EPA 744-B-97-001 Environmental Protection Prevention and Toxics June 1997 Agency (7406) Polymer Exemption Guidance Manual POLYMER EXEMPTION GUIDANCE MANUAL 5/22/97 A technical manual to accompany, but not supersede the "Premanufacture Notification Exemptions; Revisions of Exemptions for Polymers; Final Rule" found at 40 CFR Part 723, (60) FR 16316-16336, published Wednesday, March 29, 1995 Environmental Protection Agency Office of Pollution Prevention and Toxics 401 M St., SW., Washington, DC 20460-0001 Copies of this document are available through the TSCA Assistance Information Service at (202) 554-1404 or by faxing requests to (202) 554-5603. TABLE OF CONTENTS LIST OF EQUATIONS............................ ii LIST OF FIGURES............................. ii LIST OF TABLES ............................. ii 1. INTRODUCTION ............................ 1 2. HISTORY............................... 2 3. DEFINITIONS............................. 3 4. ELIGIBILITY REQUIREMENTS ...................... 4 4.1. MEETING THE DEFINITION OF A POLYMER AT 40 CFR §723.250(b)... 5 4.2. SUBSTANCES EXCLUDED FROM THE EXEMPTION AT 40 CFR §723.250(d) . 7 4.2.1. EXCLUSIONS FOR CATIONIC AND POTENTIALLY CATIONIC POLYMERS ....................... 8 4.2.1.1. CATIONIC POLYMERS NOT EXCLUDED FROM EXEMPTION 8 4.2.2. EXCLUSIONS FOR ELEMENTAL CRITERIA........... 9 4.2.3. EXCLUSIONS FOR DEGRADABLE OR UNSTABLE POLYMERS .... 9 4.2.4. EXCLUSIONS BY REACTANTS................ 9 4.2.5. EXCLUSIONS FOR WATER-ABSORBING POLYMERS........ 10 4.3. CATEGORIES WHICH ARE NO LONGER EXCLUDED FROM EXEMPTION .... 10 4.4. MEETING EXEMPTION CRITERIA AT 40 CFR §723.250(e) ....... 10 4.4.1. THE (e)(1) EXEMPTION CRITERIA............. 10 4.4.1.1. LOW-CONCERN FUNCTIONAL GROUPS AND THE (e)(1) EXEMPTION................. -
Studies on the Chemistry of Paclitaxel
STUDIES ON THE CHEMISTRY OF PACLITAXEL Haiqing Yuan Dissertation submitted to the Faculty of the Virginia Polytechnic Institute and State University in the partial fulfillment of the requirement for the degree of Doctor of Philosophy in Chemistry Dr. David G. I. Kingston, Chair Dr. Michael Calter Dr. Neal Castagnoli, Jr. Dr. Richard Gandour Dr. Larry Taylor August 11, 1998 Blacksburg, Virginia Keywords: Paclitaxel, Taxol®, synthesis, analog, SAR Copyright 1998, Haiqing Yuan STUDIES ON THE CHEMISTRY OF PACLITAXEL HAIQING YUAN (ABSTRACT) Paclitaxel is a natural occurring diterpene alkaloid originally isolated from the bark of Taxus brevifolia. It is now one of the most important chemotherapeutic agents for clinical treatment of ovarian and breast cancers. Recent clinical trials have also shown paclitaxel’s potential for the treatment of non-small-cell lung cancer, head and neck cancer, and other types of cancers. While tremendous chemical research efforts have been made in the past years, which established the fundamental structure-activity relationships of the paclitaxel molecule, and provided analogs for biochemical studies to elucidate the precise mechanism of action and for the development of second-generation agents, many areas remain to be explored. In continuation of our efforts in the structure-activity relationships study of A- norpaclitaxel, five new analogs modified at the C-1 substituent and analogs with expanded B-ring or contracted C-ring have now been prepared. Preliminary biological studies indicated that the volume rather than functionality at the C-1 position plays a role in determining the anticancer activity by controlling the relative position of the tetracyclic ring system, which in turn controls the positions of the most critical functionalities such as the C-2 benzoyl, the C-4 acetate, and the C-13 side chain. -
Organic Chemistry Tests for Hydroxyl Group
Chemistry Organic Chemistry Tests for Hydroxyl Group General Aim Method Identication of aliphatic alcohols through the chemical Detection of the presence of hydroxyl groups in detection of hydroxyl groups. aliphatic alcohols using special chemical tests. Learning Objectives (ILOs) Dene and determine aliphatic alcohols theoretically through their chemical structure. Classify organic compounds containing hydroxyl groups into aliphatic and aromatic. Compare between alcohols and other functional groups in terms of chemical structures, properties and reactions. Identify aliphatic alcohols experimentally. Select the appropriate reagents to dierentiate between alcohols and other organic compounds. Theoretical Background/Context - Aliphatic alcohols are non-aromatic hydrocarbons possessing at least one hydroxyl group within their structure. They can be either cyclic or acyclic compounds. Alcohols are considered to be neutral compounds. Aliphatic Cyclic Alcohol Aliphatic Acyclic Alcohol - Alcohols are also classied to primary, secondary and tertiary alcohols according to the number of carbon atoms attached to the carbon atom linked to the hydroxyl group. First: Preparation of Aliphatic Alcohols - Alcohols can be prepared through some chemical routes such as reduction of the corresponding aldehydes and ketones using some reducing agents such as lithium aluminum hydride or sodium borohydride. They can be also obtained from hydration of the corresponding alkenes. Some primary alcohols can be synthesized through the nucleophilic substitution of corresponding alkyl halides using potassium or sodium hydroxides. - Alcohols can be also obtained through some biological routes such as ethanol and butanol through fermentation processes in presence of glucose. Glucose is obtained from starch hydrolysis in the presence of yeast. 1 www.praxilabs.com Theoretical Background/Context (Cont’) Second: General Properties of Aliphatic Alcohols - Aliphatic alcohols are polar compounds and can form hydrogen bonds easily. -
Ganic Compounds
6-1 SECTION 6 NOMENCLATURE AND STRUCTURE OF ORGANIC COMPOUNDS Many organic compounds have common names which have arisen historically, or have been given to them when the compound has been isolated from a natural product or first synthesised. As there are so many organic compounds chemists have developed rules for naming a compound systematically, so that it structure can be deduced from its name. This section introduces this systematic nomenclature, and the ways the structure of organic compounds can be depicted more simply than by full Lewis structures. The language is based on Latin, Greek and German in addition to English, so a classical education is beneficial for chemists! Greek and Latin prefixes play an important role in nomenclature: Greek Latin ½ hemi semi 1 mono uni 1½ sesqui 2 di bi 3 tri ter 4 tetra quadri 5 penta quinque 6 hexa sexi 7 hepta septi 8 octa octo 9 ennea nona 10 deca deci Organic compounds: Compounds containing the element carbon [e.g. methane, butanol]. (CO, CO2 and carbonates are classified as inorganic.) See page 1-4. Special characteristics of many organic compounds are chains or rings of carbon atoms bonded together, which provides the basis for naming, and the presence of many carbon- hydrogen bonds. The valency of carbon in organic compounds is 4. Hydrocarbons: Compounds containing only the elements C and H. Straight chain hydrocarbons are named according to the number of carbon atoms: CH4, methane; C2H6 or H3C-CH3, ethane; C3H8 or H3C-CH2-CH3, propane; C4H10 or H3C-CH2- CH2-CH3, butane; C5H12 or CH3CH2CH2CH2CH3, pentane; C6H14 or CH3(CH2)4CH3, hexane; C7H16, heptane; C8H18, octane; C9H20, nonane; C10H22, CH3(CH2)8CH3, decane. -
Preparation of Hydroxy Aromatic Carboxylic Acids and Ester Derivatives Thereof
Europiisches Patentamt European Patent Office © Publication number: 0 049 616 Office europeen des brevets A1 EUROPEAN PATENT APPLICATION © Application number: 81304567.1 © int. ci.3: C 07 C 69/88 C 07 C 67/36 © Date of filing: 02.10.81 © Priority: 06.10.80 US 194201 © Applicant: CELANESE CORPORATION 1211 Avenue Of The Americas New York New York 10036IUS) © Date of publication of application: 14.04.82 Bulletin 82/15 © Inventor: McGinnes, James L 236 Raritan Avenue © Designated Contracting States: Middlesex New Jersey(US) BE DE FR GB IT NL © Inventor: Conciatori, Anthony B. 27. Orchard Road Chatham New Jersey(US) @ Representative: De Minvielle-Devaux, Ian Benedict Peter et al, CARPMAELS & RANSFORD 43, Bloomsbury Square London WC1 A 2RA(GB) © Preparation of hydroxy aromatic carboxylic acids and ester derivatives thereof. A process for preparing hydroxy aromatic carboxylic acids, or the ester derivatives thereof, comprises carbonylat- ing a hydroxy aromatic halide in the presence of a reactive alcohol solvent and a catalytic amount of a Group VIII metal catalyst. The process has particular applicability to the preparation of 6-hydroxy-2-naphthoic acid from 6-bromo-2- naphthol, which can be easily prepared from β-naphthol, a readily available and inexpensive starting material. This invention relates to a novel process for pre- paring hydroxy aromatic carboxylic acids or the corresponding ester derivatives thereof. This invention relates particular- ly to a novel process for the preparation of esters of hydroxy aromatic carboxylic acids such as 6-hydroxy-2- naphthoic acid by the carbonylation of a hydroxy aromatic halide, followed if desired by conversion of the esters to the acids. -
Lab Safety Manual and the Laboratory-Specific Information in the CHP Easily
Laboratory Safety Manual With the Lab-Specific Chemical Hygiene Plan Template The University of Tennessee Knoxville Campus September 2015 PURPOSE OF THE LABORATORY SAFETY MANUAL 1) Provide laboratories with useful recommendations that can help achieve compliance with the OSHA Lab Standard and workplace safety rules and regulations. 2) Equip researchers to develop a Lab-Specific Chemical Hygiene Plan Throughout this document, areas where regulatory or University requirements exist will be clearly identified using words such as “must”, “required”, “shall”, and “it is the responsibility”, etc. All other information provided within this document serves as recommendations that Environmental Health and Safety (EHS) encourages laboratories to follow as best management practices. To take advantage of the Internet, this document is formatted to be a “front door” to other resources, including useful web links. Where appropriate, web links are provided that can be clicked to view the webpage. This Laboratory Safety Manual should be considered a living document and will be reviewed at least annually and updated with your participation, comments, and suggestions. Policy Statement It is the policy of the University of Tennessee at Knoxville (UTK) to provide a safe working and learning environment. The Environmental, Health, and Safety Department has developed this manual as a guidance document to familiarize UTK faculty, staff, students, volunteers, and visitors with the campus-wide policies and procedures for the safe use of hazardous chemicals and other hazardous materials at the University. When these policies and procedures are followed, the risk of occupational exposures to chemicals and physical hazards as well as the risk of accidental environmental release of hazardous materials is minimized. -
Changxia Yuan Baran Group Meeting 4/5/2014 Commercial Available
Baran Group Meeting Changxia Yuan 4/5/2014 Commercial available peroxides* Inorganic peroxides Na2O2 CaO2 Li2O2 BaO2 Ni2O3 NiO2 xH2O H2O2(30%) ZnO2 NaBO3 4H2O MgO2 TbO2 SrO2 Na2CO3 1.5H2O sodium calcium lithium barium nickel nickel(II) hydrogen zinc sodium magnesium terbium Stronium sodium peroxide peroxide peroxide peroxide peroxide peroxide peroxide peroxide perborate peroxide peroxide peroxide percarbonate $ 109/100g $ 27/100g $ 32 /50g $ 146/500g $ 106/5g hydrate $ 350/4L $ 75/1kg tetrahydrate complex $ 30/1g $ 38/100g $ 91/ 2.5kg $ 40/ 1g $94/1kg $ 40/250g + (NH4)2S2O8 Na2S2O8 K2S2O8 2K2SO5 KHSO4 K2SO4 5[Bu4N ] SO5] HSO4] SO4] ammonium sodium potassium Oxone® OXONE® persulfate persulfate persulfate monopersulfate tetrabutylammonium salt $ 39/ 100g $ 87/ 2.5kg $ 70/ 500g compound $ 156/ 25g $ 60/ 1kg Organic peroxides-1 O CO3H O HOO tBu O O OO O CH2(CH2)9CH3 O H3C(H2C)9H2C O O tBu tBuOOH urea H2O2 BzOOBz OOH O Cl O tert-Butyl Urea Benzoyl mCPBA Cyclobutane 2-Butanone tert-Butyl Lauoyl hydroperoxide hydrogen peroxide $ 81/100g maloyl peroxide peroxide peroxide solution (5-6 M) peroxide $ 92/500g peroxide $ 129/500mL $ 134/1L $ 81/100g $ 47/25mL $ 88/ 250g $ 100/1g Cl O O Cl O Me Me O Me Me Me Me O O O O Ph O O O O OO O Me O Me O Ph O O tBu Cl Ph Me Me Me O Me 2,4-Dichlorobenzoyl Cl tert-Butyl tert-Butyl peracetate solution, Dicumyl tert-Butylperoxy 1,1-Bis(tert-amylperoxy)cyclohexane peroxide, 50% in DBP peroxybenzoate 50% in mineral spirits peroxide 2-ethylhexyl solution, 80% in mineral spirits $ 59/100g $ 86/500mL $ 77/500mL $ 123/500g -
Towards the Synthesis of the Emestrin Family of Natural Products
Towards the Synthesis of the Emestrin Family of Natural Products Brendan James Fisher ORCID: 0000-0003-0090-8951 Submitted in total fulfilment of the requirements of the degree of Doctor of Philosophy September 2018 School of Chemistry Bio21 Institute The University of Melbourne Abstract A Cope rearrangement of a vinyl pyrrole epoxide (397) was utilised to form the dihydrooxepino[4,3-b]pyrrole core (398) of the emestrin family of natural products which involved the first examples of the dearomatisation of pyrrole in this type of rearrangement. It was found that an electron withdrawing ester substituent on the C2 position of the epoxide was essential for the [3,3]-rearrangement to occur. The vinyl pyrrole epoxides were synthesised in an efficient manner by a vinylogous Darzens reaction. Density functional calculations showed lower transition state energies for Cope rearrangements of epoxides with C2 esters when compared to the unsubstituted substrates which agreed with the observed experimental results. Silyl substituted vinyl bromide esters also participated in the Darzens reactions to give the desired vinyl pyrrole epoxides in good to excellent yields. Only the triethoxysilyl vinyl epoxide 313c underwent Cope rearrangement to provide the fully substituted emestrin core dihydrooxepine. The anion derived from an aryl bromosulfone did not give the Darzens product but underwent a previously unobserved stereoselective trimerization to afford the cyclohexene 343 as a single diastereoisomer. A mechanistic rationale involving SN2’ additions, [3,3]-Cope rearrangements and a stereoselective intramolecular conjugate addition was proposed and this was supported by density functional theory (DFT) calculations. A four-step total synthesis of biaryl ether natural product violaceic acid (11) is described. -
The Role of Acid Catalysis in the Baeyer−Villiger Reaction. a Theoretical Study Robert D
Article pubs.acs.org/joc The Role of Acid Catalysis in the Baeyer−Villiger Reaction. A Theoretical Study Robert D. Bach* Department of Chemistry and Biochemistry, University of Delaware, Newark, Delaware 19716, United States *S Supporting Information ABSTRACT: Quantum mechanical calculations at the B3LYP/6- 311+G(d,p) level have examined the overall mechanism of the Baeyer−Villiger (BV) reaction with peroxyacetic acid. A series of reactions that include both the addition step and the subsequent alkyl group migration step included ketones, acetone, t-butyl methyl ketone, acetophenone, cyclohexyl methyl ketone, and cyclohexyl phenyl ketone. The combined data suggested that the first step for addition of the peroxyacetic acid oxidation catalyst to the ketone carbonyl to produce the Criegee or tetrahedral intermediate is rate- limiting and has activation barriers that range from 38 to 41 kcal/ mol without the aid of a catalyst. The rate of addition is markedly reduced by the catalytic action of a COOH functionality acting as a donor−acceptor group affecting both its proton transfer to the ketone CO oxygen in concert with transfer of the OOH proton to the carboxylic acid carbonyl. The second or alkyl group migration step has a much reduced activation barrier, and its rate is not markedly influenced by acid catalysis. The rate of both steps in the BV reaction is greatly influenced by the catalytic action of very strong acids. ■ INTRODUCTION mechanism for addition to the carbonyl group and how the hemiperacetal hydrogen migrates to the carboxylate to produce The Baeyer−Villiger (BV) reaction remains an important the final products, an ester functionality and a carboxylic acid. -
Reaction-Of-Alkenes.Pdf
8 Reactions of Alkenes Goals for Chapter 8 1 Explain why electrophilic additions are among the most common reactions of alkenes. femoral component 2 Predict the products of the reactions of alkenes, including the orientation of the reaction (regio- polyethylene bearing chemistry) and the stereochemistry. tibial plate 3 Propose mechanisms to explain the observed products of alkene reactions. 4 Use retrosynthetic analysis to solve multistep synthesis problems with alkenes as reagents, intermediates, or products. ◀ Polyethylene provides a self-lubricating surface for movement of the metal parts of an artificial knee replacement. The highly cross-linked polyethylene used in these implants is fabricated to be exceptionally tough: It wears only about 0.1 mm per year. Polyethylene is compatible with the human body, and in most cases, it does not cause a foreign body reaction even after years of constant movement in the joint. The alkene double bond is a gateway functional group. Alkene reactions lead to many other functional groups that lay the foundation for the rest of your study of organic chemistry. You can convert alkenes to alkyl halides, epoxides, alcohols, aldehydes, ketones, carboxylic acids, and other functional groups. The reactions of alkenes arise from the reactivity of their carbon–carbon double bonds. Organic chemists enjoy the challenge of taking a simple carbon–carbon double bond and manipulating it in all possible ways to produce other compounds, often mimicking biological reactions that occur in cells. This chapter covers the most common alkene reactions, including their mechanisms, reactivity, orientation, and stereochemistry. Most reactions of alkenes involve addition of atoms or groups across the double bond, with one atom or group adding to each end.