The Hpr Protein of the Phosphotransferase System
Total Page:16
File Type:pdf, Size:1020Kb
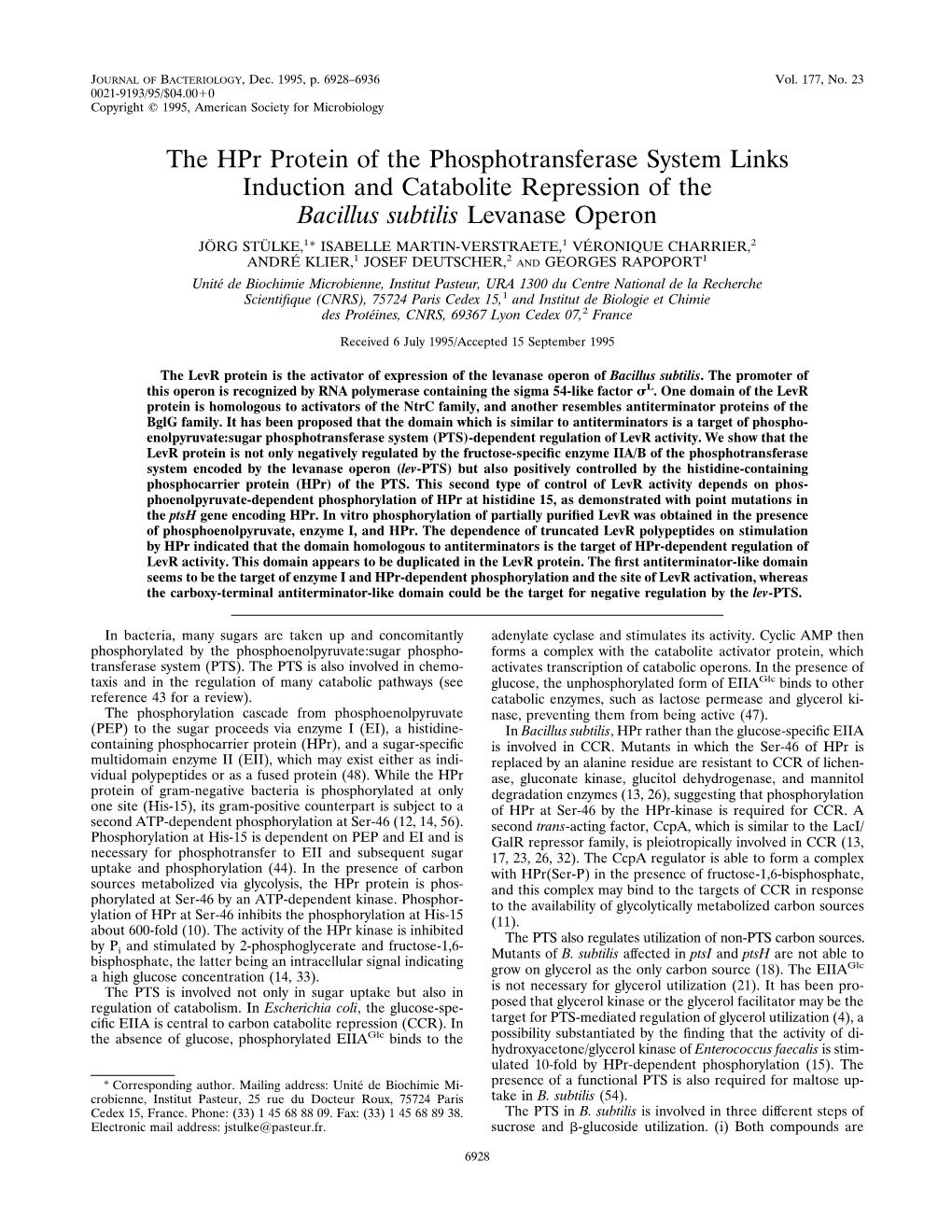
Load more
Recommended publications
-
2010 Physical Biosciences Research Meeting
2010 Physical Biosciences Research Meeting Sheraton Inner Harbor Hotel Baltimore, MD October 17-20, 2010 Office of Basic Energy Sciences Chemical Sciences, Geosciences & Biosciences Division 2010 Physical Biosciences Research Meeting Program and Abstracts Sheraton Inner Harbor Hotel Baltimore, MD October 17-20, 2010 Chemical Sciences, Geosciences, and Biosciences Division Office of Basic Energy Sciences Office of Science U.S. Department of Energy i Cover art is taken from the public domain and can be found at: http://commons.wikimedia.org/wiki/File:Blue_crab_on_market_in_Piraeus_-_Callinectes_sapidus_Rathbun_20020819- 317.jpg This document was produced under contract number DE-AC05-060R23100 between the U.S. Department of Energy and Oak Ridge Associated Universities. The research grants and contracts described in this document are, unless specifically labeled otherwise, supported by the U.S. DOE Office of Science, Office of Basic Energy Sciences, Chemical Sciences, Geosciences, and Biosciences Division. ii Foreword This volume provides a record of the 2nd biennial meeting of the Principal Investigators (PIs) funded by the Physical Biosciences program, and is sponsored by the Chemical Sciences, Geosciences, and Biosciences Division of the Office of Basic Energy Sciences (BES) in the U.S. Department of Energy (DOE). Within DOE-BES there are two programs that fund basic research in energy-relevant biological sciences, Physical Biosciences and Photosynthetic Systems. These two Biosciences programs, along with a strong program in Solar Photochemistry, comprise the current Photo- and Bio- Chemistry Team. This meeting specifically brings together under one roof all of the PIs funded by the Physical Biosciences program, along with Program Managers and staff not only from DOE-BES, but also other offices within DOE, the national labs, and even other federal funding agencies. -
Highly Thermostable and Alkaline Α-Amylase from a Halotolerant- Alkaliphilic Bacillus Sp. Ab68
Brazilian Journal of Microbiology (2008) 39:547-553 ISSN 1517-8382 HIGHLY THERMOSTABLE AND ALKALINE α-AMYLASE FROM A HALOTOLERANT- ALKALIPHILIC BACILLUS SP. AB68 Ashabil Aygan1*; Burhan Arikan2; Hatice Korkmaz2; Sadik Dinçer2; Ömer Çolak2 1Kahramanmaras Sutcu Imam University, Faculty of Science and Letters, Department of Biology, K. Maras, Turkey; 2Cukurova University, Faculty of Science and Letters, Department of Biology, Molecular Biology Laboratory, Adana, Turkey Submitted: August 13, 2007; Returned to authors for corrections: October 22, 2007; Approved: July 16, 2008. ABSTRACT An alkaliphilic and highly thermostable α-amylase producing Bacillus sp. was isolated from Van soda lake. Enzyme synthesis occurred at temperatures between 25ºC and 40ºC. Analysis of the enzyme by SDS-PAGE revealed a single band which was estimated to be 66 kDa. The enzyme was active in a broad temperature range, between 20ºC and 90ºC, with an optimum at 50ºC; and maximum activity was at pH 10.5. The enzyme was almost completely stable up to 80ºC with a remaining activity over 90% after 30 min pre-incubation. Thermostability was not increased in the presence of Ca2+. An average of 75% and 60ºC of remaining activity was observed when the enzyme was incubated between pH 5 and 9 for 1 h and for 2 h, respectively. The activity of the enzyme was inhibited by SDS and EDTA by 38% and 34%, respectively. Key words: Bacillus sp., α-amylase, Alkaliphilic, Thermostable, Enzyme. INTRODUCTION unique, buffered haloalkaline habitat appropriate for a stable development of obligately (halo)alkaliphilic microorganisms Amylases are one of the most important industrial enzymes. growing optimally at pH around 10 (39). -
(12) United States Patent (10) Patent No.: US 9,689,046 B2 Mayall Et Al
USOO9689046B2 (12) United States Patent (10) Patent No.: US 9,689,046 B2 Mayall et al. (45) Date of Patent: Jun. 27, 2017 (54) SYSTEM AND METHODS FOR THE FOREIGN PATENT DOCUMENTS DETECTION OF MULTIPLE CHEMICAL WO O125472 A1 4/2001 COMPOUNDS WO O169245 A2 9, 2001 (71) Applicants: Robert Matthew Mayall, Calgary (CA); Emily Candice Hicks, Calgary OTHER PUBLICATIONS (CA); Margaret Mary-Flora Bebeselea, A. et al., “Electrochemical Degradation and Determina Renaud-Young, Calgary (CA); David tion of 4-Nitrophenol Using Multiple Pulsed Amperometry at Christopher Lloyd, Calgary (CA); Lisa Graphite Based Electrodes', Chem. Bull. “Politehnica” Univ. Kara Oberding, Calgary (CA); Iain (Timisoara), vol. 53(67), 1-2, 2008. Fraser Scotney George, Calgary (CA) Ben-Yoav. H. et al., “A whole cell electrochemical biosensor for water genotoxicity bio-detection”. Electrochimica Acta, 2009, 54(25), 6113-6118. (72) Inventors: Robert Matthew Mayall, Calgary Biran, I. et al., “On-line monitoring of gene expression'. Microbi (CA); Emily Candice Hicks, Calgary ology (Reading, England), 1999, 145 (Pt 8), 2129-2133. (CA); Margaret Mary-Flora Da Silva, P.S. et al., “Electrochemical Behavior of Hydroquinone Renaud-Young, Calgary (CA); David and Catechol at a Silsesquioxane-Modified Carbon Paste Elec trode'. J. Braz. Chem. Soc., vol. 24, No. 4, 695-699, 2013. Christopher Lloyd, Calgary (CA); Lisa Enache, T. A. & Oliveira-Brett, A. M., "Phenol and Para-Substituted Kara Oberding, Calgary (CA); Iain Phenols Electrochemical Oxidation Pathways”, Journal of Fraser Scotney George, Calgary (CA) Electroanalytical Chemistry, 2011, 1-35. Etesami, M. et al., “Electrooxidation of hydroquinone on simply prepared Au-Pt bimetallic nanoparticles'. Science China, Chem (73) Assignee: FREDSENSE TECHNOLOGIES istry, vol. -
Studies Into the Structural Basis of the DNA Uridine Endonuclease Activity of Exonuclease III Homolog Mth212
Studies into the structural basis of the DNA uridine endonuclease activity of exonuclease III homolog Mth212 Dissertation zur Erlangung des Doktorgrades der Mathematisch-Naturwissenschaftlichen Fakultäten der Georg-August Universität zu Göttingen Vorgelegt von Khaliun Tseden aus Greifswald, Deutschland Göttingen 2011 D7 Referent: Prof. Dr. Hans-Joachim Fritz Korreferent: PD Dr. Wilfried Kramer Tag der mündlichen Prüfung: 02. Mai 2011 TABLE OF CONTENTS Table of Contents 1 Introduction ………………………………………………………………….. 1 1.1. Background to the study ………………………………………………… 1 1.1.1. Necessity of mutation avoidance………………………………… 1 1.1.2. Mutations arising in DNA during replication …………………… 1 1.1.3. Exogenous sources of DNA damage …………………………….. 2 1.1.4. Endogenous sources of DNA damage …………………………… 2 1.1.4.1. Hydrolytic DNA deamination ........……………………… 4 1.1.5. Repair of uracil in DNA …………………………………………. 5 1.1.5.1. Uracil-initiated base excision repair …………………...... 5 1.1.5.2. Uracil-initiated nucleotide incision repair ……………… 7 1.2. Objective and methodology of the study ………………………………… 9 1.2.1. Objective of the study.…………………………………………… 9 1.2.2. Methodology of the study ………………………………………. 9 1.2.2.1. Necessity of screening or selection methodology in 11 directed evolution of enzymes …………………………………………… 1.2.2.2. Selection of a protein with acquired DNA uridine 11 endonuclease activity ………………………………………………….... 2. Materials and Methods ………………………………………………………. 13 2.1. Materials …………………………………………………………………. 13 2.1.1. Bacterial strains ………………………………………………….. 13 2.1.1.1. Escherichia coli …………………………………………. 13 2.1.1.2. Bacillus subtilis ………………………………………… 14 2.1.2. Bacteriophage strains …………………………………………….. 14 2.1.3. Plasmid vectors ………………………………………………… 15 2.1.4. 2’ Desoxyriboseoligonucleotides ………………………………. 17 2.1.5. Molecular ladders and markers ………………………………….. 21 2.1.6. -
How Nature Can Exploit Nonspecific Catalytic and Carbohydrate Binding
How nature can exploit nonspecific catalytic and carbohydrate binding modules to create enzymatic specificity Fiona Cuskina,b,1, James E. Flinta,1, Tracey M. Glosterc,1,2, Carl Morlanda, Arnaud Basléa, Bernard Henrissatd, Pedro M. Coutinhod, Andrea Strazzullie, Alexandra S. Solovyovaa, Gideon J. Daviesc, and Harry J. Gilberta,b,3 aInstitute for Cell and Molecular Biosciences, The Medical School, Newcastle University, Newcastle upon Tyne NE2 4HH, United Kingdom; bThe Complex Carbohydrate Research Center, The University of Georgia, Athens, GA 30602; cStructural Biology Laboratory, Department of Chemistry, University of York, York YO10 5DD, United Kingdom; dArchitecture et Fonction des Macromolécules Biologiques, Aix-Marseille Université, Centre National de la Recherche Scientifique, Unité Mixte de Recherche 7257, 13288 Marseille Cedex 9, France; and eInstitute of Protein Biochemistry, Consiglio Nazionale delle Ricerche, 80131 Naples, Italy Edited by Arnold L. Demain, Drew University, Madison, NJ, and approved October 30, 2012 (received for review July 16, 2012) Noncatalytic carbohydrate binding modules (CBMs) are components CBMs enhance the activity of their cognate enzymes, and though of glycoside hydrolases that attack generally inaccessible substrates. the mechanism(s) by which this occurs remains uncertain, CBMs CBMs mediate a two- to fivefold elevation in the activity of endo- most likely fulfill a targeting function by increasing the effective acting enzymes, likely through increasing the concentration of the concentration of the appended enzymes, in the vicinity of the appended enzymes in the vicinity of the substrate. The function of substrate, thereby enhancing catalytic efficiency (8, 9). CBMs appended to exo-acting glycoside hydrolases is unclear Fructans, such as inulin and levan (polymers of predominantly because their typical endo-binding mode would not fulfill a target- β-2,1– or β-2,6–linked fructose units, respectively) are common ing role. -
154581A0.Pdf
No. 3914, NOVEMBER 4, 1944 NATURE 581 Alleged Role of Fructofuranose in the ture of fructofuranoses and fructopyranoses. The recent demonstration that glucose-1-phosphate (Cori Synthesis of Levan ester) and fructose form a dynamic equilibrium with THE view was long widely entertained that cells sucrose and phosphoric acid in the presence of a. synthesize macromolecules of polysaccharides and specific enzyme8 corroborates this view. Addition of proteins by a reversion .of the process of hydrolysis. fructose to sucrose does not inhibit levan production It has been suggested accordingly that the synthesis from the latter, yet fructose itself, although it pre of the polyfructoside levan specifically from aldo sumably contains ready fructofuranose, is not con side< >fructofuranosides (sucrose, raffinose) involves verted into levan by levansucrase7 • Similarly, levan two distinct steps : first, hydrolysis of the substrate ; sucrase fails to form levan from reaction mixtures secondly, polymerization of fructofuranose b;r a con in which fructofuranose is sustainedly liberated in densation involving removal of water1• Bacteria statu nascendi, for example, in reaction mixtures of which form levan from sucrose do so also from methyl gamma fructoside + yeast invertase, and of raffinose•. This polymerative type of sucrose de inulin inulase. gradation is concurrent with an ordinary hydrolytic (c) Extracts of an Aerobacter, although they pro inversions·'· The same banteria ferment !evans. duce levan from sucrose and hydro'yse the latter as Investigators might be tempted by these correlations well, do not hydrolyse levan. Thus they contain to consider the enzyme system, levansucrase, to be levansucrase and invertase but no polyfructosidase but a mixture of invertase and polyfructosidase. -
First Crystal Structure of an Endo- Levanase – the BT1760 from A
www.nature.com/scientificreports Corrected: Author Correction OPEN First crystal structure of an endo- levanase – the BT1760 from a human gut commensal Bacteroides Received: 5 April 2019 Accepted: 24 May 2019 thetaiotaomicron Published online: 11 June 2019 Karin Ernits 1, Priit Eek 2, Tiit Lukk 2, Triinu Visnapuu 1 & Tiina Alamäe 1 The endo-levanase BT1760 of a human gut commensal Bacteroides thetaiotaomicron randomly cuts a β-2,6-linked fructan, levan, into fructo-oligosaccharides providing a prebiotic substrate for gut microbiota. Here we introduce the crystal structure of BT1760 at resolution of 1.65 Å. The fold of the enzyme is typical for GH32 family proteins: a catalytic N-terminal fve-bladed β-propeller connected with a C-terminal β-sandwich domain. The levantetraose-bound structure of catalytically inactive mutant E221A at 1.90-Å resolution reveals diferences in substrate binding between the endo-acting fructanases. A shallow substrate-binding pocket of the endo-inulinase INU2 of Aspergillus fcuum binds at least three fructose residues at its fat bottom. In the levantetraose-soaked crystal of the endo-levanase E221A mutant the ligand was bent into the pond-like substrate pocket with its fructose residues making contacts at −3, −2, −1 and + 1 subsites residing at several pocket depths. Binding of levantetraose to the β-sandwich domain was not detected. The N- and C-terminal modules of BT1760 did not bind levan if expressed separately, the catalytic domain lost its activity and both modules tended to precipitate. We gather that endo-levanase BT1760 requires both domains for correct folding, solubility and stability of the protein. -
Substrate Recognition by Two Members of Glycoside Hydrolase Family 32 Involved in Fructo-Oligosaccharide Metabolism in Lactobacillus Acidophilus NCFM
Substrate recognition of glycoside hydrolase family 32 protein Substrate Recognition by Two Members of Glycoside Hydrolase Family 32 Involved in Fructo-oligosaccharide Metabolism in Lactobacillus acidophilus NCFM Hiroyuki NAKAI1,2*, Birte SVENSSON3, and Ken'ichi OHTSUBO1,2 (Received Decsmber 6, 2011) Summary Two fructo-oligosaccharide active enzymes, BfrA and ScrB, of glycoside hydrolase family 32 are found in Lactobacillus acidophilus NCFM and belong to phylogenetic clusters of bacterial β-fructosidase and sucrose 6-phosphate hydrolase, respectively, involved in the intracellular metabolism of kesto-oligosaccharides [β-D-fructofuranosyl-(2,1)] n-β-D-fructofuranosyl-(2,1)-α-D-glucopyranose (n, 1-3) and sucrose. Recombinant ScrB, produced in Escherichia coli, showed 20-1500-fold higher catalytic efficiency (kcat/Km) toward sucrose than raffinose [α-D-galactopyranosyl-(1,6)-α -D-glucopyranosyl-(1,2)-β-D-fructofuranose] and kesto-oligosaccharides. At high concentration of sucrose (0.4-1.0 M) ScrB catalysed formation of 1-kestose [β-D-fructofuranosyl-(2,1)-β-D-fructofuranosyl-(2,1)-α-D-glucopyranose] in 2.3-15% yield by transglycosylation. Recombinant BfrA in contrast efficiently hydrolysed kesto-oligosaccharides, in particular 1-kestose with 70- 300-fold higher kcat/Km than for sucrose and raffinose and thecat k /Km values decreased slightly with increasing degree of the polymerization of the kesto-oligosaccharides. Neither BfrA nor ScrB degraded the β-(2,1)- and β-(2,6)-linked fructans, inulin and levan. The outcome of the present detailed -
Characterization of the Streptococcus Mutans GS-5Frua Gene Encoding
INFECTION AND IMMUNITY, Nov. 1992, p. 4621-4632 Vol. 60, No. 11 0019-9567/92/114621-12$02.00/0 Copyright © 1992, American Society for Microbiology Characterization of the Streptococcus mutans GS-5 fruA Gene Encoding Exo-,1-D-Fructosidase ROBERT A. BURNEl 2* AnD JANA E. C. PENDERS' Departments ofDental Research' and ofMicrobiology and Immunology, 2 University ofRochester Medical Center, Rochester, New York 14642 Received 8 June 1992/Accepted 3 September 1992 The complete nucleotide sequence (5,010 bp) of the fructanase gene (fruA) and flanking regions of the chromosome of Streptococcus mutans GS-5 was determined. The fruA gene appears to be the sole transcript arising from a proximal promoter. The presumed precursor of the secreted FruA protein consists of 1,423 amino acids, and it has an Mr of 158,656 and a pI of 4.82. The N terminus of FruA has characteristics in common with signal peptides of gram-positive organisms. The C terminus consists of a serine- and threonine-rich region, followed by the peptide LPDTGD, 4 charged amino acids, 21 amino acids with a strongly hydrophobic character, and a charged pentapeptide tail, which are proposed to correspond to the wall- spanning region, the LPXTGX consensus sequence, and the membrane-spanning domains ofsurface-associated proteins of gram-positive cocci. The FruA protein has significant homology with the BaciUus subtilis levanase (SacC), the Bacteroidesfragilis levanase (ScrL), yeast invertases, and a number ofother 1-fructosidases but not with fructosyltransferase, glucosyltransferases, or glucan-binding proteins of oral streptococci. Genes with homology tofiuA were detected in S. mutans serotype c, e, and f strains, Streptococcus ratus, Streptococcus salivarius, and Streptococcus sanguis. -
Evidence of Cellulose Metabolism by the Giant Panda Gut Microbiome
Evidence of cellulose metabolism by the giant panda gut microbiome Lifeng Zhua,1,QiWua,1, Jiayin Daia, Shanning Zhangb, and Fuwen Weia,2 aKey Laboratory of Animal Ecology and Conservation Biology, Institute of Zoology, Chinese Academy of Sciences, Beijing 100101, China and bChina Wildlife Conservation Association, Beijing 100714, China Edited by Rita R. Colwell, University of Maryland, College Park, MD, and approved September 7, 2011 (received for review December 2, 2010) The giant panda genome codes for all necessary enzymes associ- cellulose digestion (10). Although the giant panda can use non- ated with a carnivorous digestive system but lacks genes for cellulosic material from the bamboo diet using enzymes coded in enzymes needed to digest cellulose, the principal component of its own genome, digestion of cellulose and hemicellulose is im- their bamboo diet. It has been posited that this iconic species must possible based on the panda’s genetic composition, and must be therefore possess microbial symbionts capable of metabolizing dependent on gut microbiome. However, previous research using cellulose, but these symbionts have remained undetected. Here we culture methods and small-scale sequencing identified three examined 5,522 prokaryotic ribosomal RNA gene sequences in wild predominant bacteria from the panda gut—Escherichia coli, and captive giant panda fecal samples. We found lower species Streptococcus, and Enterobacteriaceae—none of which aids in richness of the panda microbiome than of mammalian microbiomes cellulose digestion (11–13). Thus, an incomplete understanding for herbivores and nonherbivorous carnivores. We detected 13 of the gut microbial ecosystem in this interesting and high-profile operational taxonomic units closely related to Clostridium groups I species remains because of restrictions in methodology and past and XIVa, both of which contain taxa known to digest cellulose. -
Supplemental Table S1: Comparison of the Deleted Genes in the Genome-Reduced Strains
Supplemental Table S1: Comparison of the deleted genes in the genome-reduced strains Legend 1 Locus tag according to the reference genome sequence of B. subtilis 168 (NC_000964) Genes highlighted in blue have been deleted from the respective strains Genes highlighted in green have been inserted into the indicated strain, they are present in all following strains Regions highlighted in red could not be deleted as a unit Regions highlighted in orange were not deleted in the genome-reduced strains since their deletion resulted in severe growth defects Gene BSU_number 1 Function ∆6 IIG-Bs27-47-24 PG10 PS38 dnaA BSU00010 replication initiation protein dnaN BSU00020 DNA polymerase III (beta subunit), beta clamp yaaA BSU00030 unknown recF BSU00040 repair, recombination remB BSU00050 involved in the activation of biofilm matrix biosynthetic operons gyrB BSU00060 DNA-Gyrase (subunit B) gyrA BSU00070 DNA-Gyrase (subunit A) rrnO-16S- trnO-Ala- trnO-Ile- rrnO-23S- rrnO-5S yaaC BSU00080 unknown guaB BSU00090 IMP dehydrogenase dacA BSU00100 penicillin-binding protein 5*, D-alanyl-D-alanine carboxypeptidase pdxS BSU00110 pyridoxal-5'-phosphate synthase (synthase domain) pdxT BSU00120 pyridoxal-5'-phosphate synthase (glutaminase domain) serS BSU00130 seryl-tRNA-synthetase trnSL-Ser1 dck BSU00140 deoxyadenosin/deoxycytidine kinase dgk BSU00150 deoxyguanosine kinase yaaH BSU00160 general stress protein, survival of ethanol stress, SafA-dependent spore coat yaaI BSU00170 general stress protein, similar to isochorismatase yaaJ BSU00180 tRNA specific adenosine -
Cazymes Catalogue 2021
cazymes 2021 Visit the Online Store www.nzytech.com Newsletter NZYWallet Instantaneous Quotes Subscribe our newsletter to receive NZYWallet is a prepaid account Do you need an urgent quote? Just awesome news and promotions. that oers the flexibility you need add your products to Cart, proceed to focus on your research. With to Checkout, select quote and it’s NZYWallet you can buy any done! product from our Online Store, check your up-to-date balance and track your latest orders. Contact our customer service at [email protected] or your local sales representative for more information. Follow us: 2021 NZYTech NZYTech 2 Visit the Online Store www.nzytech.com Newsletter NZYWallet Instantaneous Quotes Subscribe our newsletter to receive NZYWallet is a prepaid account Do you need an urgent quote? Just awesome news and promotions. that oers the flexibility you need add your products to Cart, proceed to focus on your research. With to Checkout, select quote and it’s NZYWallet you can buy any done! product from our Online Store, check your up-to-date balance and track your latest orders. Contact our customer service at [email protected] or your local sales representative for more information. Follow us: cazymes 3 4 2021 NZYTech NZYTech OVERVIEW 8 GLYCOSIDE HYDROLASES 10 Acetylgalactosaminidases Acetylglucosaminidases Agarases Amylases @ a glance Amylomaltases Arabinanases Arabinofuranosidases Arabinopyranosidases Arabinoxylanases Carrageenases Cellobiohydrolases Cellodextrinases Cellulases Chitinases Chitosanases Dextranases Fructanases Fructofuranosidases