Functional Characterization of Peroxisomes in the Heart and the Role of Pex11α and Pex14 in Cardiomyocytes
Total Page:16
File Type:pdf, Size:1020Kb
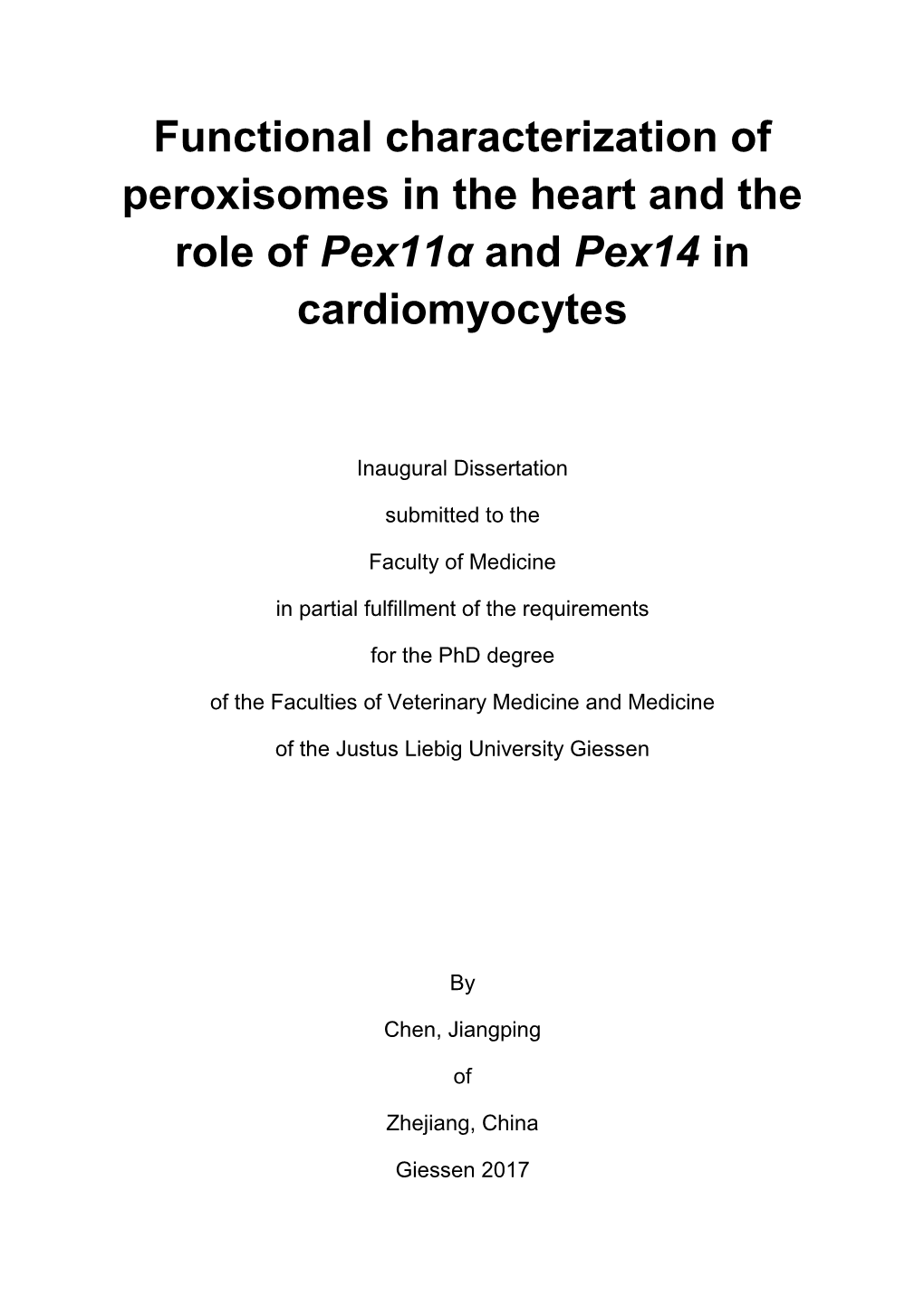
Load more
Recommended publications
-
Supplementary Table S4. FGA Co-Expressed Gene List in LUAD
Supplementary Table S4. FGA co-expressed gene list in LUAD tumors Symbol R Locus Description FGG 0.919 4q28 fibrinogen gamma chain FGL1 0.635 8p22 fibrinogen-like 1 SLC7A2 0.536 8p22 solute carrier family 7 (cationic amino acid transporter, y+ system), member 2 DUSP4 0.521 8p12-p11 dual specificity phosphatase 4 HAL 0.51 12q22-q24.1histidine ammonia-lyase PDE4D 0.499 5q12 phosphodiesterase 4D, cAMP-specific FURIN 0.497 15q26.1 furin (paired basic amino acid cleaving enzyme) CPS1 0.49 2q35 carbamoyl-phosphate synthase 1, mitochondrial TESC 0.478 12q24.22 tescalcin INHA 0.465 2q35 inhibin, alpha S100P 0.461 4p16 S100 calcium binding protein P VPS37A 0.447 8p22 vacuolar protein sorting 37 homolog A (S. cerevisiae) SLC16A14 0.447 2q36.3 solute carrier family 16, member 14 PPARGC1A 0.443 4p15.1 peroxisome proliferator-activated receptor gamma, coactivator 1 alpha SIK1 0.435 21q22.3 salt-inducible kinase 1 IRS2 0.434 13q34 insulin receptor substrate 2 RND1 0.433 12q12 Rho family GTPase 1 HGD 0.433 3q13.33 homogentisate 1,2-dioxygenase PTP4A1 0.432 6q12 protein tyrosine phosphatase type IVA, member 1 C8orf4 0.428 8p11.2 chromosome 8 open reading frame 4 DDC 0.427 7p12.2 dopa decarboxylase (aromatic L-amino acid decarboxylase) TACC2 0.427 10q26 transforming, acidic coiled-coil containing protein 2 MUC13 0.422 3q21.2 mucin 13, cell surface associated C5 0.412 9q33-q34 complement component 5 NR4A2 0.412 2q22-q23 nuclear receptor subfamily 4, group A, member 2 EYS 0.411 6q12 eyes shut homolog (Drosophila) GPX2 0.406 14q24.1 glutathione peroxidase -
Supplementary Table 2
Supplementary Table 2. Differentially Expressed Genes following Sham treatment relative to Untreated Controls Fold Change Accession Name Symbol 3 h 12 h NM_013121 CD28 antigen Cd28 12.82 BG665360 FMS-like tyrosine kinase 1 Flt1 9.63 NM_012701 Adrenergic receptor, beta 1 Adrb1 8.24 0.46 U20796 Nuclear receptor subfamily 1, group D, member 2 Nr1d2 7.22 NM_017116 Calpain 2 Capn2 6.41 BE097282 Guanine nucleotide binding protein, alpha 12 Gna12 6.21 NM_053328 Basic helix-loop-helix domain containing, class B2 Bhlhb2 5.79 NM_053831 Guanylate cyclase 2f Gucy2f 5.71 AW251703 Tumor necrosis factor receptor superfamily, member 12a Tnfrsf12a 5.57 NM_021691 Twist homolog 2 (Drosophila) Twist2 5.42 NM_133550 Fc receptor, IgE, low affinity II, alpha polypeptide Fcer2a 4.93 NM_031120 Signal sequence receptor, gamma Ssr3 4.84 NM_053544 Secreted frizzled-related protein 4 Sfrp4 4.73 NM_053910 Pleckstrin homology, Sec7 and coiled/coil domains 1 Pscd1 4.69 BE113233 Suppressor of cytokine signaling 2 Socs2 4.68 NM_053949 Potassium voltage-gated channel, subfamily H (eag- Kcnh2 4.60 related), member 2 NM_017305 Glutamate cysteine ligase, modifier subunit Gclm 4.59 NM_017309 Protein phospatase 3, regulatory subunit B, alpha Ppp3r1 4.54 isoform,type 1 NM_012765 5-hydroxytryptamine (serotonin) receptor 2C Htr2c 4.46 NM_017218 V-erb-b2 erythroblastic leukemia viral oncogene homolog Erbb3 4.42 3 (avian) AW918369 Zinc finger protein 191 Zfp191 4.38 NM_031034 Guanine nucleotide binding protein, alpha 12 Gna12 4.38 NM_017020 Interleukin 6 receptor Il6r 4.37 AJ002942 -
Genome-Wide Insights on Gastrointestinal Nematode
www.nature.com/scientificreports OPEN Genome‑wide insights on gastrointestinal nematode resistance in autochthonous Tunisian sheep A. M. Ahbara1,2, M. Rouatbi3,4, M. Gharbi3,4, M. Rekik1, A. Haile1, B. Rischkowsky1 & J. M. Mwacharo1,5* Gastrointestinal nematode (GIN) infections have negative impacts on animal health, welfare and production. Information from molecular studies can highlight the underlying genetic mechanisms that enhance host resistance to GIN. However, such information often lacks for traditionally managed indigenous livestock. Here, we analysed 600 K single nucleotide polymorphism genotypes of GIN infected and non‑infected traditionally managed autochthonous Tunisian sheep grazing communal natural pastures. Population structure analysis did not fnd genetic diferentiation that is consistent with infection status. However, by contrasting the infected versus non‑infected cohorts using ROH, LR‑GWAS, FST and XP‑EHH, we identifed 35 candidate regions that overlapped between at least two methods. Nineteen regions harboured QTLs for parasite resistance, immune capacity and disease susceptibility and, ten regions harboured QTLs for production (growth) and meat and carcass (fatness and anatomy) traits. The analysis also revealed candidate regions spanning genes enhancing innate immune defence (SLC22A4, SLC22A5, IL‑4, IL‑13), intestinal wound healing/repair (IL‑4, VIL1, CXCR1, CXCR2) and GIN expulsion (IL‑4, IL‑13). Our results suggest that traditionally managed indigenous sheep have evolved multiple strategies that evoke and enhance GIN resistance and developmental stability. They confrm the importance of obtaining information from indigenous sheep to investigate genomic regions of functional signifcance in understanding the architecture of GIN resistance. Small ruminants (sheep and goats) make immense socio-economic and cultural contributions across the globe. -
Comparative Genomics of Peroxisome Biogenesis Proteins: Making Sense of the PEX Mess
bioRxiv preprint doi: https://doi.org/10.1101/2020.12.16.423121; this version posted December 16, 2020. The copyright holder for this preprint (which was not certified by peer review) is the author/funder, who has granted bioRxiv a license to display the preprint in perpetuity. It is made available under aCC-BY 4.0 International license. Comparative genomics of peroxisome biogenesis proteins: making sense of the PEX mess Renate L.M. Jansen1,*, Carlos Santana Molina2,*, Marco van den Noort1,3, Damien P. Devos2,4 and Ida J. van der Klei1,4 1Molecular Cell Biology, Groningen Biomolecular Sciences and Biotechnology Institute, University of Groningen, 9800CC, the Netherlands 2Centro Andaluz de Biología del Desarrollo, CSIC, Universidad Pablo de Olavide, Carretera de Utrera, Km.1, Seville 41013, Spain 3Current address: Membrane Enzymology, Groningen Biomolecular Sciences and Biotechnology Institute, University of Groningen, 9800CC, the Netherlands 4Corresponding authors: [email protected], ORCID ID 0000-0001-7165-9679, P.O. Box 11103, 9700CC, Groningen, The Netherlands and [email protected], ORCID ID 0000- 0002-9453-4753, Carretera de Utrera, Km.1, Seville 41013, Spain *These authors equally contributed. bioRxiv preprint doi: https://doi.org/10.1101/2020.12.16.423121; this version posted December 16, 2020. The copyright holder for this preprint (which was not certified by peer review) is the author/funder, who has granted bioRxiv a license to display the preprint in perpetuity. It is made available under aCC-BY 4.0 International license. Abstract PEX genes encode proteins involved in peroxisome biogenesis and proliferation. Using a comparative genomics approach, we clarify the evolutionary relationships between the 37 known PEX proteins in a representative set of eukaryotes, including all common model organisms, pathogenic unicellular eukaryotes and human. -
Downloaded Per Proteome Cohort Via the Web- Site Links of Table 1, Also Providing Information on the Deposited Spectral Datasets
www.nature.com/scientificreports OPEN Assessment of a complete and classifed platelet proteome from genome‑wide transcripts of human platelets and megakaryocytes covering platelet functions Jingnan Huang1,2*, Frauke Swieringa1,2,9, Fiorella A. Solari2,9, Isabella Provenzale1, Luigi Grassi3, Ilaria De Simone1, Constance C. F. M. J. Baaten1,4, Rachel Cavill5, Albert Sickmann2,6,7,9, Mattia Frontini3,8,9 & Johan W. M. Heemskerk1,9* Novel platelet and megakaryocyte transcriptome analysis allows prediction of the full or theoretical proteome of a representative human platelet. Here, we integrated the established platelet proteomes from six cohorts of healthy subjects, encompassing 5.2 k proteins, with two novel genome‑wide transcriptomes (57.8 k mRNAs). For 14.8 k protein‑coding transcripts, we assigned the proteins to 21 UniProt‑based classes, based on their preferential intracellular localization and presumed function. This classifed transcriptome‑proteome profle of platelets revealed: (i) Absence of 37.2 k genome‑ wide transcripts. (ii) High quantitative similarity of platelet and megakaryocyte transcriptomes (R = 0.75) for 14.8 k protein‑coding genes, but not for 3.8 k RNA genes or 1.9 k pseudogenes (R = 0.43–0.54), suggesting redistribution of mRNAs upon platelet shedding from megakaryocytes. (iii) Copy numbers of 3.5 k proteins that were restricted in size by the corresponding transcript levels (iv) Near complete coverage of identifed proteins in the relevant transcriptome (log2fpkm > 0.20) except for plasma‑derived secretory proteins, pointing to adhesion and uptake of such proteins. (v) Underrepresentation in the identifed proteome of nuclear‑related, membrane and signaling proteins, as well proteins with low‑level transcripts. -
Obesity Is Associated with Macrophage Accumulation
Obesity is associated with See the related Commentary beginning on page 1785. macrophage accumulation in adipose tissue Stuart P. Weisberg,1 Daniel McCann,1 Manisha Desai,2 Michael Rosenbaum,1 Rudolph L. Leibel,1,3,4 and Anthony W. Ferrante, Jr.3,4 1Division of Molecular Genetics, Department of Pediatrics, 2Department of Biostatistics, 3Deparment of Medicine, and 4Naomi Berrie Diabetes Center, Columbia University, New York, New York, USA Obesity alters adipose tissue metabolic and endocrine function and leads to an increased release of fatty acids, hormones, and proinflammatory molecules that contribute to obesity associated compli- cations. To further characterize the changes that occur in adipose tissue with increasing adiposity, we profiled transcript expression in perigonadal adipose tissue from groups of mice in which adiposity varied due to sex, diet, and the obesity-related mutations agouti (Ay) and obese (Lepob). We found that the expression of 1,304 transcripts correlated significantly with body mass. Of the 100 most significantly correlated genes, 30% encoded proteins that are characteristic of macrophages and are positively cor- related with body mass. Immunohistochemical analysis of perigonadal, perirenal, mesenteric, and sub- cutaneous adipose tissue revealed that the percentage of cells expressing the macrophage marker F4/80 (F4/80+) was significantly and positively correlated with both adipocyte size and body mass. Similar relationships were found in human subcutaneous adipose tissue stained for the macrophage antigen CD68. Bone marrow transplant studies and quantitation of macrophage number in adipose tissue from macrophage-deficient (Csf1op/op) mice suggest that these F4/80+ cells are CSF-1 dependent, bone marrow–derived adipose tissue macrophages. -
Downloaded from [22]
BMC Genomics BioMed Central Research article Open Access Cell array-based intracellular localization screening reveals novel functional features of human chromosome 21 proteins Yu-Hui Hu1,2, Hans-Jörg Warnatz1, Dominique Vanhecke1, Florian Wagner3, Andrea Fiebitz1, Sabine Thamm1, Pascal Kahlem1,4, Hans Lehrach1, Marie- Laure Yaspo1 and Michal Janitz*1 Address: 1Department of Vertebrate Genomics, Max Planck Institute for Molecular Genetics, 14195 Berlin, Germany, 2FU Berlin, Department of Biology, Chemistry and Pharmacy, 14195 Berlin, Germany, 3RZPD German Resource Center for Genome Research, 14059 Berlin, Germany and 4Department of Hematology, Oncology, and Tumor Immunology, Humboldt University, Charite, Berlin, Germany Email: Yu-Hui Hu - [email protected]; Hans-Jörg Warnatz - [email protected]; Dominique Vanhecke - [email protected]; Florian Wagner - [email protected]; Andrea Fiebitz - [email protected]; Sabine Thamm - [email protected]; Pascal Kahlem - [email protected]; Hans Lehrach - [email protected]; Marie- Laure Yaspo - [email protected]; Michal Janitz* - [email protected] * Corresponding author Published: 16 June 2006 Received: 13 March 2006 Accepted: 16 June 2006 BMC Genomics 2006, 7:155 doi:10.1186/1471-2164-7-155 This article is available from: http://www.biomedcentral.com/1471-2164/7/155 © 2006 Hu et al; licensee BioMed Central Ltd. This is an Open Access article distributed under the terms of the Creative Commons Attribution License (http://creativecommons.org/licenses/by/2.0), which permits unrestricted use, distribution, and reproduction in any medium, provided the original work is properly cited. Abstract Background: Trisomy of human chromosome 21 (Chr21) results in Down's syndrome, a complex developmental and neurodegenerative disease. -
Fatty Liver Due to Increased De Novo Lipogenesis: Alterations in the Hepatic Peroxisomal Proteome
fcell-07-00248 October 24, 2019 Time: 16:7 # 1 ORIGINAL RESEARCH published: 25 October 2019 doi: 10.3389/fcell.2019.00248 Fatty Liver Due to Increased de novo Lipogenesis: Alterations in the Hepatic Peroxisomal Proteome Birgit Knebel1,2*, Pia Fahlbusch1,2, Matthias Dille1,2, Natalie Wahlers1,2, Sonja Hartwig1,2, Sylvia Jacob1,2, Ulrike Kettel1,2, Martina Schiller1,2, Diran Herebian3, Cornelia Koellmer1,2, Stefan Lehr1,2, Dirk Müller-Wieland4 and Jorg Kotzka1,2 1 Leibniz Center for Diabetes Research, Institute of Clinical Biochemistry and Pathobiochemistry, German Diabetes Center at the Heinrich-Heine-University Duesseldorf, Düsseldorf, Germany, 2 German Center for Diabetes Research (DZD), Partner Duesseldorf, Düsseldorf, Germany, 3 Department of General Pediatrics, Neonatology and Pediatric Cardiology, Medical Faculty, University Children’s Hospital, Heinrich-Heine-University Düsseldorf, Düsseldorf, Germany, 4 Department of Internal Medicine I, Clinical Research Centre, University Hospital Aachen, Aachen, Germany In non-alcoholic fatty liver disease (NAFLD) caused by ectopic lipid accumulation, lipotoxicity is a crucial molecular risk factor. Mechanisms to eliminate lipid overflow can prevent the liver from functional complications. This may involve increased secretion of lipids or metabolic adaptation to ß-oxidation in lipid-degrading organelles such as mitochondria and peroxisomes. In addition to dietary factors, increased plasma fatty Edited by: acid levels may be due to increased triglyceride synthesis, lipolysis, as well as de novo -
Comparative Analysis of Gene Regulation by the Transcription Factor Ppara Between Mouse and Human
Comparative Analysis of Gene Regulation by the Transcription Factor PPARa between Mouse and Human Maryam Rakhshandehroo1,2, Guido Hooiveld1,2, Michael Mu¨ ller1,2, Sander Kersten1,2* 1 Nutrigenomics Consortium, Top Institute (TI) Food and Nutrition, Wageningen, the Netherlands, 2 Nutrition, Metabolism and Genomics group, Division of Human Nutrition, Wageningen University, Wageningen, the Netherlands Abstract Background: Studies in mice have shown that PPARa is an important regulator of hepatic lipid metabolism and the acute phase response. However, little information is available on the role of PPARa in human liver. Here we set out to compare the function of PPARa in mouse and human hepatocytes via analysis of target gene regulation. Methodology/Principal Findings: Primary hepatocytes from 6 human and 6 mouse donors were treated with PPARa agonist Wy14643 and gene expression profiling was performed using Affymetrix GeneChips followed by a systems biology analysis. Baseline PPARa expression was similar in human and mouse hepatocytes. Depending on species and time of exposure, Wy14643 significantly induced the expression of 362–672 genes. Surprisingly minor overlap was observed between the Wy14643-regulated genes from mouse and human, although more substantial overlap was observed at the pathway level. Xenobiotics metabolism and apolipoprotein synthesis were specifically regulated by PPARa in human hepatocytes, whereas glycolysis-gluconeogenesis was regulated specifically in mouse hepatocytes. Most of the genes commonly regulated in mouse and human were involved in lipid metabolism and many represented known PPARa targets, including CPT1A, HMGCS2, FABP1, ACSL1, and ADFP. Several genes were identified that were specifically induced by PPARa in human (MBL2, ALAS1, CYP1A1, TSKU) or mouse (Fbp2, lgals4, Cd36, Ucp2, Pxmp4). -
Identification of Novel Regulatory Genes in Acetaminophen
IDENTIFICATION OF NOVEL REGULATORY GENES IN ACETAMINOPHEN INDUCED HEPATOCYTE TOXICITY BY A GENOME-WIDE CRISPR/CAS9 SCREEN A THESIS IN Cell Biology and Biophysics and Bioinformatics Presented to the Faculty of the University of Missouri-Kansas City in partial fulfillment of the requirements for the degree DOCTOR OF PHILOSOPHY By KATHERINE ANNE SHORTT B.S, Indiana University, Bloomington, 2011 M.S, University of Missouri, Kansas City, 2014 Kansas City, Missouri 2018 © 2018 Katherine Shortt All Rights Reserved IDENTIFICATION OF NOVEL REGULATORY GENES IN ACETAMINOPHEN INDUCED HEPATOCYTE TOXICITY BY A GENOME-WIDE CRISPR/CAS9 SCREEN Katherine Anne Shortt, Candidate for the Doctor of Philosophy degree, University of Missouri-Kansas City, 2018 ABSTRACT Acetaminophen (APAP) is a commonly used analgesic responsible for over 56,000 overdose-related emergency room visits annually. A long asymptomatic period and limited treatment options result in a high rate of liver failure, generally resulting in either organ transplant or mortality. The underlying molecular mechanisms of injury are not well understood and effective therapy is limited. Identification of previously unknown genetic risk factors would provide new mechanistic insights and new therapeutic targets for APAP induced hepatocyte toxicity or liver injury. This study used a genome-wide CRISPR/Cas9 screen to evaluate genes that are protective against or cause susceptibility to APAP-induced liver injury. HuH7 human hepatocellular carcinoma cells containing CRISPR/Cas9 gene knockouts were treated with 15mM APAP for 30 minutes to 4 days. A gene expression profile was developed based on the 1) top screening hits, 2) overlap with gene expression data of APAP overdosed human patients, and 3) biological interpretation including assessment of known and suspected iii APAP-associated genes and their therapeutic potential, predicted affected biological pathways, and functionally validated candidate genes. -
SUPPLEMENTARY MATERIALS and METHODS PBMC Transcriptomics
BMJ Publishing Group Limited (BMJ) disclaims all liability and responsibility arising from any reliance Supplemental material placed on this supplemental material which has been supplied by the author(s) Gut SUPPLEMENTARY MATERIALS AND METHODS PBMC transcriptomics identifies immune-metabolism disorder during the development of HBV-ACLF Contents l Supplementary methods l Supplementary Figure 1 l Supplementary Figure 2 l Supplementary Figure 3 l Supplementary Figure 4 l Supplementary Figure 5 l Supplementary Table 1 l Supplementary Table 2 l Supplementary Table 3 l Supplementary Table 4 l Supplementary Tables 5-14 l Supplementary Table 15 l Supplementary Table 16 l Supplementary Table 17 Li J, et al. Gut 2021;0:1–13. doi: 10.1136/gutjnl-2020-323395 BMJ Publishing Group Limited (BMJ) disclaims all liability and responsibility arising from any reliance Supplemental material placed on this supplemental material which has been supplied by the author(s) Gut SUPPLEMENTARY METHODS Test for HBV DNA The levels of HBV DNA were detected using real-time PCR with a COBAS® AmpliPrep/COBAS® TaqMan 48 System (Roche, Basel, Switzerland) and HBV Test v2.0. Criteria for diagnosing cirrhosis Pathology The gold standard for the diagnosis of cirrhosis is a liver biopsy obtained through a percutaneous or transjugular approach.1 Ultrasonography was performed 2-4 hours before biopsy. Liver biopsy specimens were obtained by experienced physicians. Percutaneous transthoracic puncture of the liver was performed according to the standard criteria. After biopsy, patients were monitored in the hospital with periodic analyses of haematocrit and other vital signs for 24 hours. Cirrhosis was diagnosed according to the globally agreed upon criteria.2 Cirrhosis is defined based on its pathological features under a microscope: (a) the presence of parenchymal nodules, (b) differences in liver cell size and appearance, (c) fragmentation of the biopsy specimen, (d) fibrous septa, and (d) an altered architecture and vascular relationships. -
Lipid Droplets and Peroxisomes: Key Players in Cellular Lipid Homeostasis Or a Matter of Fat—Store ’Em up Or Burn ’Em Down
YEASTBOOK CELL STRUCTURE & TRAFFICKING Lipid Droplets and Peroxisomes: Key Players in Cellular Lipid Homeostasis or A Matter of Fat—Store ’em Up or Burn ’em Down Sepp D. Kohlwein,*,1 Marten Veenhuis,† and Ida J. van der Klei†,1 *Institute of Molecular Biosciences, University of Graz, 8010 Graz, Austria, and yMolecular Cell Biology, University of Groningen, 9700CC Groningen, The Netherlands ABSTRACT Lipid droplets (LDs) and peroxisomes are central players in cellular lipid homeostasis: some of their main functions are to control the metabolic flux and availability of fatty acids (LDs and peroxisomes) as well as of sterols (LDs). Both fatty acids and sterols serve multiple functions in the cell—as membrane stabilizers affecting membrane fluidity, as crucial structural elements of membrane- forming phospholipids and sphingolipids, as protein modifiers and signaling molecules, and last but not least, as a rich carbon and energy source. In addition, peroxisomes harbor enzymes of the malic acid shunt, which is indispensable to regenerate oxaloacetate for gluconeogenesis, thus allowing yeast cells to generate sugars from fatty acids or nonfermentable carbon sources. Therefore, failure of LD and peroxisome biogenesis and function are likely to lead to deregulated lipid fluxes and disrupted energy homeostasis with detrimental consequences for the cell. These pathological consequences of LD and peroxisome failure have indeed sparked great biomedical interest in understanding the biogenesis of these organelles, their functional roles in lipid homeostasis, interaction with cellular metabolism and other organelles, as well as their regulation, turnover, and inheritance. These questions are particularly burning in view of the pandemic development of lipid-associated disorders worldwide.