Analysis of Heavy Ion Fusion Targets
Total Page:16
File Type:pdf, Size:1020Kb
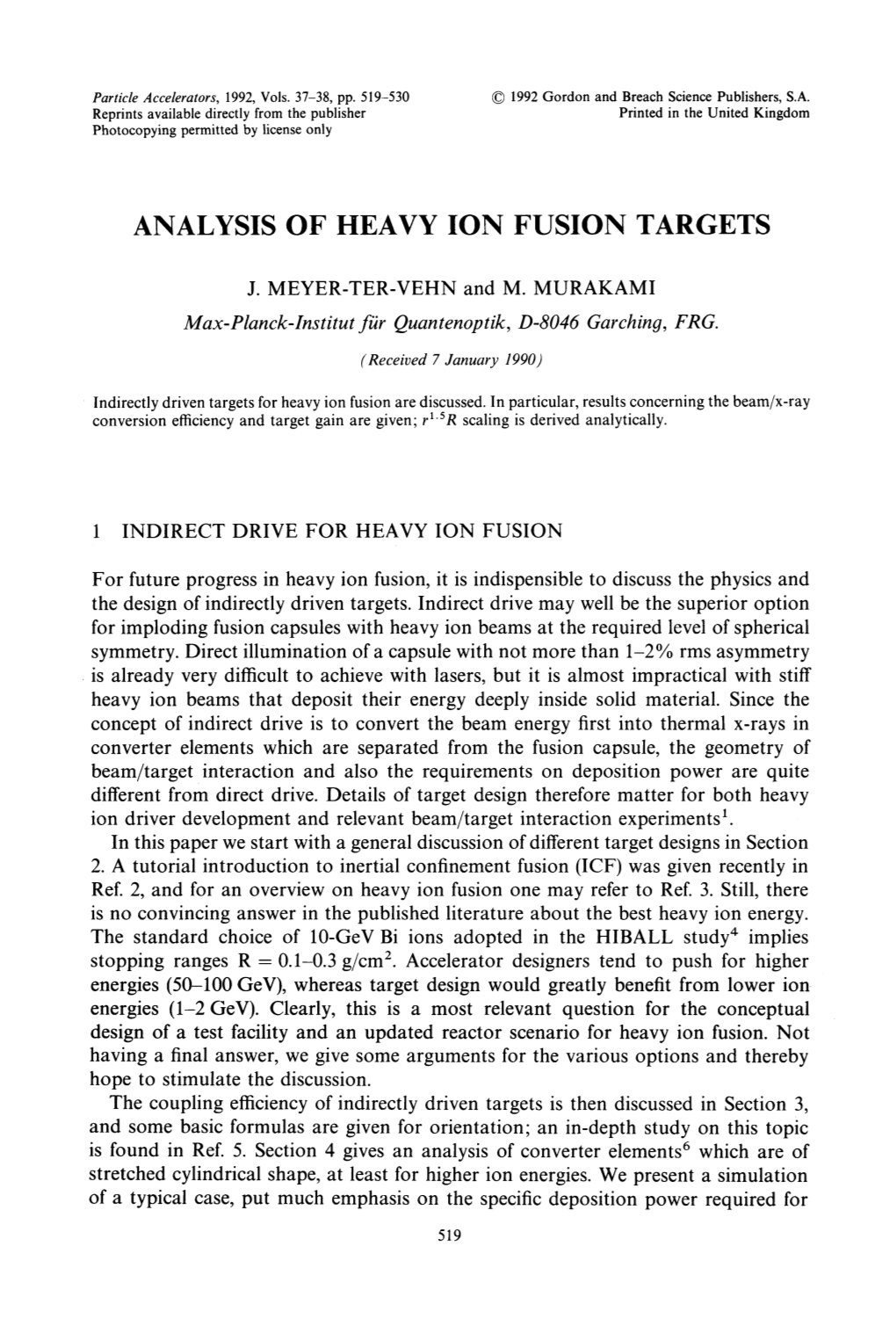
Load more
Recommended publications
-
1958 Geneva Conference on the Discontinuation of Nuclear Weapons
1 2 3 4 5 6 7 8 Presidential Decisions on Stockpile Stewardship Test-Based Stewardship and the Cold War Transitioning to Stockpile Stewardship Science: Research, Development, and Technology Deterrence and the Life Extension Program Global Nuclear Security Timeline of U.S. Stockpile Stewardship Innovation The Path Forward Today, I am announcing my “decision to negotiate a true zero-yield comprehensive test ban.” U.S. President Bill Clinton, August 11, 1995 The National Defense Authorization Act for fiscal year 1994 (P.L. 103-160) estab- lished the Stockpile Stewardship Program (SSP) to sustain the nuclear deterrent in the absence of nuclear explosive testing. The SSP supports U.S. national security missions through leading-edge scientific, engineering, and technical tools and expertise – a U.S. response to the end of the Cold War and the need to remake the global nuclear landscape. One year later, on August 11, 1995, Presi- dent Bill Clinton announced that the United States would support a “zero yield” Compre- hensive Nuclear-Test-Ban Treaty (CTBT): “I am assured by the Secretary of Energy and the Directors of our nu- clear weapons labs that we can meet the challenge of maintaining our nuclear deterrent under a Compre- hensive Test-Ban Treaty through a Science-Based Stockpile Stewardship program without nuclear testing.” This year, the Nation and the Department of Energy (DOE) celebrate the 20th anniver- sary of that announcement and the scientific and technical capabilities that have devel- oped to support this policy direction. The national investment in stockpile stewardship has enabled resolution of many stockpile issues and provided more detailed knowledge than what could have been attained through nuclear explosive testing. -
Memorandum to Participants JASON 1994 Summer Study
Hydronnclear Testing and the Comprehensive Test Ban: Memorandum to Participants JASON 1994 Summer Study Natural Resources Defense Council, Inc. 1350 New York Avenue, NW, Suite 300 Washington, D.C. 20005 Tel (main): (202) 783-7800 Cochran (direct dial): (202) 624-9329 Paine (direct dial): (202) 624-9350 Fax: (202) 783-5917 INTERNET: [email protected] Although no commonly accepted defInition exists, a "hydronuclear test" may be generally described as a nuclear weapons test, or high-explosive driven criticality experiment, characterized by a nuclear energy release that is insuffIcient to heat the core material to the plasma temperatures that would cause it to explode "like a bomb." In such a test, the TNT equivalent energy release from fission would therefore not exceed the amount released by the chemical high explosive used to compress the fIssile material, and could be considerably ,less. Nuclear-weapon states, and possibly other states, also perform high-explosive driven implosion experiments using fusjon material, although" these' are not nonnally characterized as "hydronuclear tests". Hydronuclear tests can serve a useful role in the development of the full spectrum of unboosted fIssion weapons, including'first generation nuclear weapons of the implosion type with yields in the 5 to 30 kiloton range, more sophisticated designs with yields up to about a megaton, and advanced micro-nuclear weapons with yields of 5 to 500 tons. For pure fIssion weapons hydronuclear tests can be used to:' * optimize the timing of initiation of the -
General Fusion
General Fusion Fusion Power Associates, 2011 Annual Meeting 1 General Fusion Making commercially viable fusion power a reality. • Founded in 2002, based in Vancouver, Canada • Plan to demonstrate a fusion system capable of “net gain” within 3 years • Industrial and institutional partners including Los Alamos National Lab and the Canadian Government • $32.5M in venture capital, $4.5M in government support Fusion Power Associates, 2011 Annual Meeting 2 General Fusion’s Acoustically Driven MTF Fusion Power Associates, 2011 Annual Meeting 3 Commercialization Advantages Fusion Challenge General Fusion Solution 1.5 m of liquid lead lithium greatly lowers the neutron energy spectrum Neutron activation and embrittlement of structure Low neutron load at the metal wall Low activation Low radiation damage n,2n reaction in lead 4π coverage Tritium breeding Thick blanket High tritium breeding ratio of 1.6 Heat extraction Heat extraction by the working fluid Pb -Li Solubility of tritium in Pb -Li is low Tritium safety 100 M W plant size Low tritium inventory (2g) Pneumatic energy storage >100X lower System cost cost than capacitors Cost of targets in pulsed Liquid metal compression systems - “kopeck” problem No consumables Fusion Power Associates, 2011 Annual Meeting 4 Development Plan 4 years PHASE I Proof of principle Completed 2009 PHASE IIa Construct key components at full scale 2.5 years Prove system can be built $30M Progress to Date Plasma compression tests PHASE II 2012 PHASE IIb 2 years Demonstration of Net Gain Build net gain prototype $35M -
Yol 2 7 Ns 1 0 Barc/1995/P/005 O O 5 Government of India 6 Atomic Energy Commission
TRN-IN9600313 S BARC/i995)!>/005 CO I> NUCLEAR PHYSICS DIVISION BIENNIAL REPORT 1993-1994 Edited by K. Kumar and S. K. Kataria 1995 YOL 2 7 NS 1 0 BARC/1995/P/005 O O 5 GOVERNMENT OF INDIA 6 ATOMIC ENERGY COMMISSION U 0! NUCLEAR PHYSICS DIVISION BIENNIAL REPORT 1993-1994 Edited by: K. Kumar and S.K. Kataria Nuclear Physics Division BHABHA ATOMIC RESEARCH CENTRE BOMBAY, INDIA 1995 BARC/1993/P/003 BIBLIOGRAPHIC DESCRIPTION SHEET FOR TECHNICAL REPORT (as p»r IS t 9400 - 1980) 01 Security classification t Unclassified 02 Distribution : External 03 Report status t New 04 Series 3 BARC External 03 Report type : Progress Report 06 Report No. : BARC/1995/P/005 07 Part No. or Volume No. t 08 Contract No. s 10 Title and subtitle i Nuclear Physics Division biennial report 1993-1994 11 Collation t 93 p., figs., tabs. 13 Project No. : 2O Personal author (s) i K. Kumar; S.K. Kataria (eds.) 21 Affiliation of author (s) i Nuclear Physics Division, Bhabha Atomic Research Centre, Bombay 22 Corporate author(s) i Bhabha Atomic Research Centre, Bombay-400 083 23 Originating unit s Nuclear Physics Division, BARC, Bombay 24 Sponsor(s) Name i Department of Atomic Energy Type i Government 30 Date of submission s August 1993 31 Publication/Issue date September 1995 ccntd...(ii> (ii) 40 Publisher/Distributor i Head, Library and Information Division, Bhabha Atomic Research Centre, Bombay 42 Form of distribution i Hard Copy 90 Language of text i English 91 Language of summary i English 92 No. -
Nuclear Fusion Power – an Overview of History, Present and Future
International Journal of Advanced Network, Monitoring and Controls Volume 04, No.04, 2019 Nuclear Fusion Power – An Overview of History, Present and Future Stewart C. Prager Department of Physics University of Wisconsin – Madison Madison, WI 53706, USA E-mail: [email protected] Summary—Fusion power offers the prospect of an allowing the nuclei to fuse together. Such conditions almost inexhaustible source of energy for future can occur when the temperature increases, causing the generations, but it also presents so far insurmountable ions to move faster and eventually reach speeds high engineering challenges. The fundamental challenge is to enough to bring the ions close enough together. The achieve a rate of heat emitted by a fusion plasma that nuclei can then fuse, causing a release of energy. exceeds the rate of energy injected into the plasma. The main hope is centered on tokamak reactors and II. FUSION TECHNOLOGY stellarators which confine deuterium-tritium plasma In the Sun, massive gravitational forces create the magnetically. right conditions for fusion, but on Earth they are much Keywords-Fusion Energy; Hydrogen Power; Nuclear Fusion harder to achieve. Fusion fuel – different isotopes of hydrogen – must be heated to extreme temperatures of I. INTRODUCTION the order of 50 million degrees Celsius, and must be Today, many countries take part in fusion research kept stable under intense pressure, hence dense enough to some extent, led by the European Union, the USA, and confined for long enough to allow the nuclei to Russia and Japan, with vigorous programs also fuse. The aim of the controlled fusion research underway in China, Brazil, Canada, and Korea. -
Compact Fusion Reactors
Compact fusion reactors Tomas Lind´en Helsinki Institute of Physics 26.03.2015 Fusion research is currently to a large extent focused on tokamak (ITER) and inertial confinement (NIF) research. In addition to these large international or national efforts there are private companies performing fusion research using much smaller devices than ITER or NIF. The attempt to achieve fusion energy production through relatively small and compact devices compared to tokamaks decreases the costs and building time of the reactors and this has allowed some private companies to enter the field, like EMC2, General Fusion, Helion Energy, Lockheed Martin and LPP Fusion. Some of these companies are trying to demonstrate net energy production within the next few years. If they are successful their next step is to attempt to commercialize their technology. In this presentation an overview of compact fusion reactor concepts is given. CERN Colloquium 26th of March 2015 Tomas Lind´en (HIP) Compact fusion reactors 26.03.2015 1 / 37 Contents Contents 1 Introduction 2 Funding of fusion research 3 Basics of fusion 4 The Polywell reactor 5 Lockheed Martin CFR 6 Dense plasma focus 7 MTF 8 Other fusion concepts or companies 9 Summary Tomas Lind´en (HIP) Compact fusion reactors 26.03.2015 2 / 37 Introduction Introduction Climate disruption ! ! Pollution ! ! ! Extinctions Ecosystem Transformation Population growth and consumption There is no silver bullet to solve these issues, but energy production is "#$%&'$($#!)*&+%&+,+!*&!! central to many of these issues. -.$&'.$&$&/!0,1.&$'23+! Economically practical fusion power 4$(%!",55*6'!"2+'%1+!$&! could contribute significantly to meet +' '7%!89 !)%&',62! the future increased energy :&(*61.'$*&!(*6!;*<$#2!-.=%6+! production demands in a sustainable way. -
Dangerous Thermonuclear Quest: the Potential of Explosive Fusion Research for the Development of Pure Fusion Weapons
Dangerous Thermonuclear Quest: The Potential of Explosive Fusion Research for the Development of Pure Fusion Weapons Arjun Makhijani, Ph.D. Hisham Zerriffi July 1998 Minor editing revisions made in 2003. Table of Contents Preface i Summary and Recommendations v Summary of Findings vi Recommendations vii Chapter 1: Varieties of Nuclear Weapons 1 A. Historical Background 1 B. Converting Matter into Energy 4 C. Fission energy 5 D. Fusion energy 8 E. Fission-fusion weapons 16 Chapter 2: Inertial Confinement Fusion Basics 19 A. Deposition of driver energy 24 B. Driver requirements 25 C. Fuel pellet compression 27 D. Thermonuclear ignition 29 Chapter 3: Various ECF Schemes 31 A. Laser Drivers 32 B. Ion Beam Drivers 34 1. Heavy Ion Beams 35 a. Induction Accelerators 36 b. Radio-Frequency Accelerators 36 2. Light Ion Beams 37 C. Z-pinch 39 D. Chemical Explosives 40 E. Advanced materials manufacturing 42 1. Nanotechnology 42 2. Metallic Hydrogen 45 Chapter 4: The Prospects for Pure Fusion Weapons 47 A. Requirements for pure fusion weapons 47 B. Overall assessment of non-fission-triggered nuclear weapons 48 1. Ignition 48 2. Drivers 53 C. Overall technical prognosis for non-fission triggered nuclear weapons 54 D. Fusion power and fusion weapons - comparative requirements 56 Chapter 5: Nuclear Disarmament and Non-Proliferation Issues Related to Explosive Confinement Fusion 59 A. The Science Based Stockpile Stewardship Program 62 1. Reliability 62 a. Reliability definition 63 b. Future reliability problems 64 c. Relevance of NIF to reliability of the current stockpile 65 2. The US laser fusion program as a weapons development program 65 3. -
A Novel Three-Axis Cylindrical Hohlraum Designed
www.nature.com/scientificreports OPEN A novel three-axis cylindrical hohlraum designed for inertial confinement fusion ignition Received: 19 May 2016 Longyu Kuang1,2,3, Hang Li1,2,3, Longfei Jing1, Zhiwei Lin1, Lu Zhang1, Liling Li1, Accepted: 12 September 2016 Yongkun Ding1,2,3,4, Shaoen Jiang1,2,3,4, Jie Liu3,4,5 & Jian Zheng2,4 Published: 05 October 2016 A novel ignition hohlraum for indirect-drive inertial confinement fusion is proposed, which is named three-axis cylindrical hohlraum (TACH). TACH is a kind of 6 laser entrance holes (LEHs) hohlraum, which is orthogonally jointed of three cylindrical hohlraums. Laser beams are injected through every entrance hole with the same incident angle of 55°. A view-factor simulation result shows that the time-varying drive asymmetry of TACH is less than 1.0% in the whole drive pulse period without any supplementary technology. Coupling efficiency of TACH is close to that of 6 LEHs spherical hohlraum with corresponding size. Its plasma-filling time is close to that of typical cylindrical ignition hohlraum. Its laser plasma interaction has as low backscattering as the outer cone of the cylindrical ignition hohlraum. Therefore, TACH combines most advantages of various hohlraums and has little predictable risk, providing an important competitive candidate for ignition hohlraum. In indirect-drive Inertial Confinement Fusion (ICF), laser beams are injected into a high-Z hohlraum through laser entrance holes (LEHs) and are converted into X-ray radiation, then the radiation irradiates a low-Z capsule in the center of the hohlraum to bring the central fuel in the capsule to ignition conditions1–6. -
Signature Redacted %
EXAMINATION OF THE UNITED STATES DOMESTIC FUSION PROGRAM ARCHW.$ By MASS ACHUSETTS INSTITUTE Lauren A. Merriman I OF IECHNOLOLGY MAY U6 2015 SUBMITTED TO THE DEPARTMENT OF NUCLEAR SCIENCE AND ENGINEERING I LIBR ARIES IN PARTIAL FULFILLMENT OF THE REQUIREMENTS FOR THE DEGREE OF BACHELOR OF SCIENCE IN NUCLEAR SCIENCE AND ENGINEERING AT THE MASSACHUSETTS INSTITUTE OF TECHNOLOGY FEBRUARY 2015 Lauren A. Merriman. All Rights Reserved. - The author hereby grants to MIT permission to reproduce and to distribute publicly Paper and electronic copies of this thesis document in whole or in part. Signature of Author:_ Signature redacted %. Lauren A. Merriman Department of Nuclear Science and Engineering May 22, 2014 Signature redacted Certified by:. Dennis Whyte Professor of Nuclear Science and Engineering I'l f 'A Thesis Supervisor Signature redacted Accepted by: Richard K. Lester Professor and Head of the Department of Nuclear Science and Engineering 1 EXAMINATION OF THE UNITED STATES DOMESTIC FUSION PROGRAM By Lauren A. Merriman Submitted to the Department of Nuclear Science and Engineering on May 22, 2014 In Partial Fulfillment of the Requirements for the Degree of Bachelor of Science in Nuclear Science and Engineering ABSTRACT Fusion has been "forty years away", that is, forty years to implementation, ever since the idea of harnessing energy from a fusion reactor was conceived in the 1950s. In reality, however, it has yet to become a viable energy source. Fusion's promise and failure are both investigated by reviewing the history of the United States domestic fusion program and comparing technological forecasting by fusion scientists, fusion program budget plans, and fusion program budget history. -
Nuclear Weapons Journal PO Box 1663 Mail Stop A142 Los Alamos, NM 87545 WEAPONS Issue 2 • 2009 Journal
Presorted Standard U.S. Postage Paid Albuquerque, NM nuclear Permit No. 532 Nuclear Weapons Journal PO Box 1663 Mail Stop A142 Los Alamos, NM 87545 WEAPONS Issue 2 • 2009 journal Issue 2 2009 LALP-10-001 Nuclear Weapons Journal highlights ongoing work in the nuclear weapons programs at Los Alamos National Laboratory. NWJ is an unclassified publication funded by the Weapons Programs Directorate. Managing Editor-Science Writer Editorial Advisor Send inquiries, comments, subscription requests, Margaret Burgess Jonathan Ventura or address changes to [email protected] or to the Designer-Illustrator Technical Advisor Nuclear Weapons Journal Jean Butterworth Sieg Shalles Los Alamos National Laboratory Mail Stop A142 Science Writers Printing Coordinator Los Alamos, NM 87545 Brian Fishbine Lupe Archuleta Octavio Ramos Los Alamos National Laboratory, an affirmative action/equal opportunity employer, is operated by Los Alamos National Security, LLC, for the National Nuclear Security Administration of the US Department of Energy under contract DE-AC52-06NA25396. This publication was prepared as an account of work sponsored by an agency of the US Government. Neither Los Alamos National Security, LLC, the US Government nor any agency thereof, nor any of their employees make any warranty, express or implied, or assume any legal liability or responsibility for the accuracy, completeness, or usefulness of any information, apparatus, product, or process disclosed or represent that its use would not infringe privately owned rights. Reference herein to any specific commercial product, process, or service by trade name, trademark, manufacturer, or otherwise does not necessarily constitute or imply its endorsement, recommendation, or favoring by Los Alamos National Security, LLC, the US Government, or any agency thereof. -
Accelerators for Heavy Ion Inertial Fusion: Progress and Plans*
IAEA-F1-CN-60 ACCELERATORS FOR HEAVY ION INERTIAL FUSION: PROGRESS AND PLANS* R. 0. BANGERTER Lawrence Berkeley Laboratory Berkeley, California A. FRIEDMAN Lawrence Livermore National Laboratory Livermore, California W. B. HERRMANNSFELDT Stanford Linear Accelerator Center Stanford, California United States of America Abstract The Heavy Ion Inertia! Fusion Program is the principal part of the Inertial Fusion Energy Program in the Office of Fusion Energy of the U.S. Department of Energy. The emphasis of the Heavy Ion Program is the development of accelerators for fusion power production. Target physics research and some elements of fusion chamber development are supported in the much larger Inertial Confinement Fusion Program, a dual purpose (defense and energy) program in the Defense Programs part of the Department of Energy. The accelerator research program will establish feasibility through a sequence of scaled experiments that will demonstrate key physics and engineering issues at low cost compared to other fusion programs. This paper discusses progress in the accelerator program and outlines how the planned research will address the key economic issues of inertial fusion energy. 1. INTRODUCTION The realization of hopes that thermonuclear fusion will fulfill the energy requirements for a future when fossil fuels are no longer viable depends on finding solutions to two issues: (1) economically and environmentally attractive energy from mature power plants, and (2) an affordable research program leading to this goal. Arguably, the affordable research program is the more important issue at the present time. Section 2 describes a research program leading to a heavy-ion fusion power plant. Section 3 discusses the long- term cost of electricity and Section 4 describes current research. -
Progress of Indirect Drive Inertial Confinement Fusion in the Us
Kline DOI:10.1088/1741-4326/ab1ecf OV/2-5 Progress of Indirect Drive Inertial Confinement Fusion in the USA J. L. Kline1, S. H. Batha1, L. R. Benedetti2, D. Bennett2, S. Bhandarkar2, L. F. Berzak-Hopkines2, J. Biener2, M. M. Biener2, R. Bionta2, E. Bond2, D. Bradley2, P. A. Bradley1, T. Braun2, D. A. Callahan2, J. Caggiano2, T. Cardenas2, C. Cerjjan2, B. Cagadas2, D. Clark2, C. Castro2, W. S. Daughton1, E. L. Dewald2, T. Döppner2, L. Divol2, E. S. Dodd1, R. Dylla-Spears2, M. Eckart2, D. Edgell3, M. Farrell4, J. Field2, F. Fierro1, D. N. Fittinghoff2, M. Gatu-Johnson5, S. Johnson2, G. Grim2, N. Guler1, S. Haan2, B. M. Haines1, C. E. Hamilton1, A. V. Hamza2, E. P. Hartouni2, R. Hatarik2, K. Hernderson1, H. W. Herrmann1, D. Hinkel2, D. D. Ho2, M. Hohenburger2, D. Hoover4, H. Huang4, M. L. Hoppe4, O. A. Hurricane2, N. Izumi2, O. S. Jones2, S. Khan2, B. J. Kozioziemski2, C. Kong2, J. Kroll2, G. A. Kyrala2, R. J. Leeper2, S. LePape2, E. Loomis1, T. Ma2, A. J. Mackinnon2, A. G. MacPhee2, S. MacLaren2, L. Masse2, J. McNaney2, N. B. Meezan2, J. F. Merrill1, E. C. Merritt1, J. L. Milovich2, D. S. Montgomery1, J. Moody2, A. Nikroo2, J. Oertel1, R. E. Olson1, A. Pak2, S. Palaniyappan1, P. Patel2, B. M. Patterson1, T. S. Perry1, R. R. Peterson1, E. Piceno2, J. E. Ralph2, R. B. Randolph1, N. Rice4, H. F. Robey2, J. S. Ross2, J. R. Rygg3, M. R. Sacks1, J. Sauppe1, J. Salmonson2, D. Sayre2, J. D. Sater2, M. Schneider2, M. Schoff4, D. W. Schmidt1, S.