Mineralogy, Petrography, and Microstructural Evolution of The
Total Page:16
File Type:pdf, Size:1020Kb
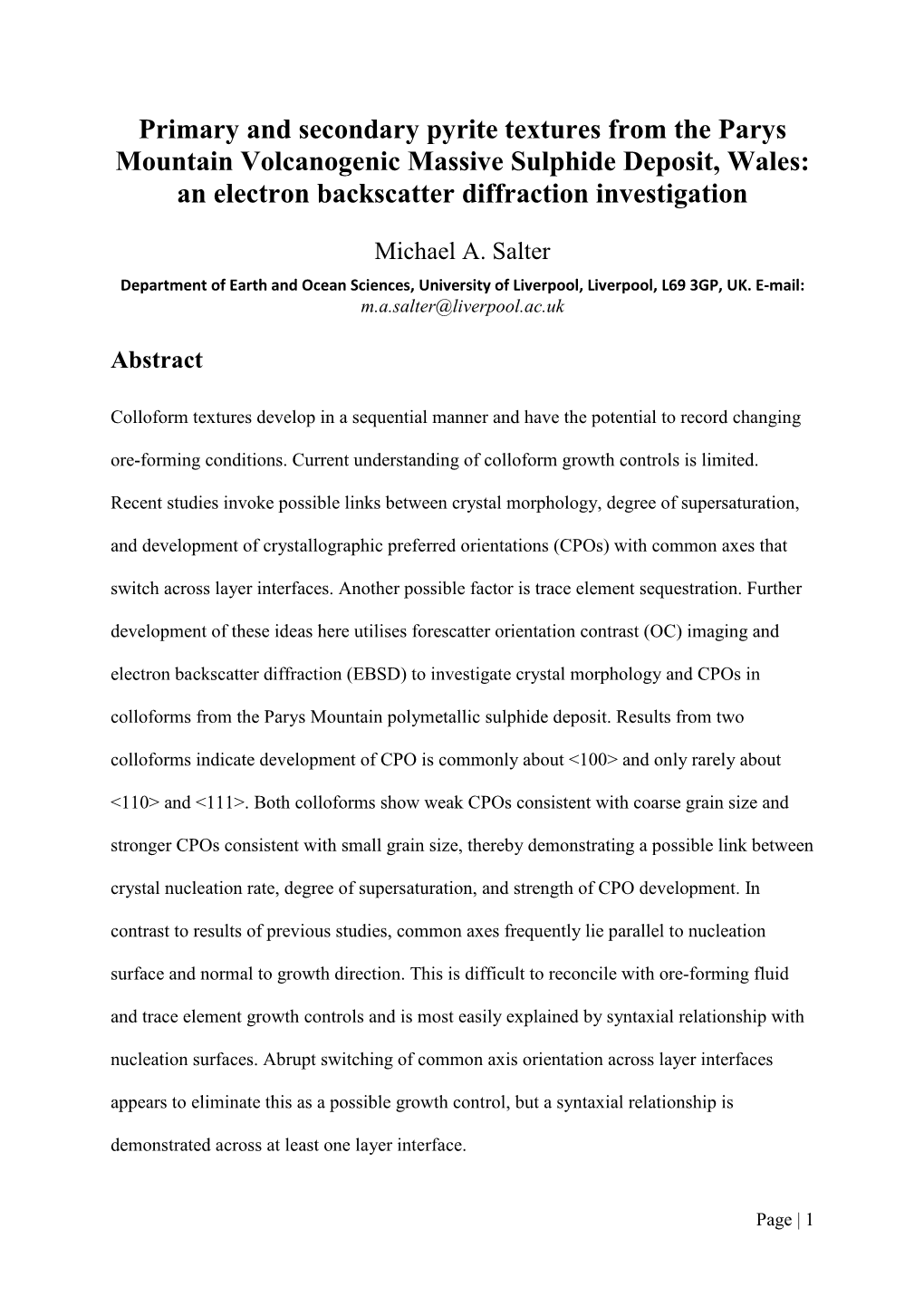
Load more
Recommended publications
-
The Smelting of Copper
Chapter 4 The Smelting of Copper The first written account of the processes of smelting and refining of copper is to be found in the 12th century.1 On smelting: Copper is engendered in the earth. When a vein of which is found, it is acquired with the greatest labour by digging and breaking. It is a stone of a green colour and most hard, and naturally mixed with lead. This stone, dug up in abundance, is placed upon a pile, and burned after the manner of chalk, nor does it change colour, but yet looses its hardness, so that it can be broken up. Then, being bruised small, it is placed in the furnace; coals and the bellows being applied, it is incessantly forged by day and night. On refining: Of the purification of copper. Take an iron dish of the size you wish, and line it inside and and out with clay strongly beaten and mixed, and it is carefully dried. Then place it before a forge upon the coals, so that when the bellows acts upon it the wind may issue partly within and partly above it, and not below it. And very small coals being placed around it equally, and add over it a heap of coals. When, by blowing a long time, this has become melted, uncover it and cast immediately fine ashes over it, and stir it with a thin and dry piece of wood as if mixing it, and you will directly see the burnt lead adhere to these ashes like a glue. -
Wales Heritage Interpretation Plan
TOUCH STONE GREAT EXPLANATIONS FOR PEOPLE AT PLACES Cadw Pan-Wales heritage interpretation plan Wales – the first industrial nation Ysgogiad DDrriivviinngg FFoorrcceess © Cadw, Welsh Government Interpretation plan October 2011 Cadw Pan-Wales heritage interpretation plan Wales – the first industrial nation Ysgogiad Driving Forces Interpretation plan Prepared by Touchstone Heritage Management Consultants, Red Kite Environment and Letha Consultancy October 2011 Touchstone Heritage Management Consultants 18 Rose Crescent, Perth PH1 1NS, Scotland +44/0 1738 440111 +44/0 7831 381317 [email protected] www.touchstone-heritage.co.uk Michael Hamish Glen HFAHI FSAScot FTS, Principal Associated practice: QuiteWrite Cadw – Wales – the first industrial nation / Interpretation plan i ____________________________________________________________________________________________________________________________________________________________________________ Contents 1 Foreword 1 2 Introduction 3 3 The story of industry in Wales 4 4 Our approach – a summary 13 5 Stakeholders and initiatives 14 6 Interpretive aim and objectives 16 7 Interpretive themes 18 8 Market and audiences 23 9 Our proposals 27 10 Interpretive mechanisms 30 11 Potential partnerships 34 12 Monitoring and evaluation 35 13 Appendices: Appendix A: Those consulted 38 Appendix B: The brief in full 39 Appendix C: National Trust market segments 41 Appendix D: Selected people and sites 42 The illustration on the cover is part of a reconstruction drawing of Blaenavon Ironworks by Michael -
Inspection Report Ysgol Gynradd Penysarn 2017
A report on Ysgol Gynradd Penysarn Penysarn Anglesey LL69 9AZ Date of inspection: October 2017 by Estyn, Her Majesty’s Inspectorate for Education and Training in Wales A report on Ysgol Gynradd Penysarn October 2017 About Ysgol Gynradd Penysarn Ysgol Gynradd Penysarn is situated approximately two miles from Amlwch on the Isle of Anglesey. Welsh is the main medium of the school’s life and work. There are 98 pupils between three and eleven years old on roll, including eight part-time nursery age children. They are divided into four mixed-age classes. Approximately 13% of pupils are eligible for free school meals. This is lower than the national percentage (19%). Approximately half the pupils come from Welsh-speaking homes, and very few are from ethnic minority backgrounds. The school has identified 22% of its pupils as having additional learning needs, but very few have a statement of special educational needs. The headteacher was appointed to the post in January 2017. He was not at the school during the inspection. The school was last inspected in January 2012. Further information is available from the Welsh Government My Local School website at the link below. http://mylocalschool.wales.gov.uk/Schools/SchoolSearch?lang=en 1 A report on Ysgol Gynradd Penysarn October 2017 Summary During their time at the school, most pupils make sound progress and achieve well. Most pupils show positive attitudes towards their work and concentrate well in lessons. On the whole, teachers provide interesting learning activities, which motivate pupils to enjoy learning. The Welsh language is at the heart of the school’s life and work, and there is a clear emphasis on ensuring that pupils have a good awareness of the local area’s history and culture. -
Michael Hughes of Sutton: the Influence of Welsh Copper On
MICHAEL HUGHES OF SUTTON THE INFLUENCE OF WELSH COPPER ON LANCASHIRE BUSINESS, 1780-1815'1 ' BY J. R. HARRIS, B.A. Read 31 March 1949 ICHAEL HUGHES of Sherdley House in the township of M Sutton was very much the sort of man who made the Indus trial Revolution. From his ledgers one can see the economic vitality of the period displayed in the varied business activities of a single influential man. His actions determined the course of local industrial and financial advance: in turn the trends of the time moulded his own economic and business life. In this paper it is intended to speak first of the connection between Welsh copper mining and the Lancashire smelting industry, and then of the investments and financial activities of Hughes. Michael Hughes (1752-1825) was the youngest child of Hugh Hughes of Lleinog in Anglesey, (2) whose family remained obscure until his eldest son, the Rev. Edward Hughes, M.A., married a lady, Mary Lewis, who inherited some land in Anglesey, (3) particularly half a barren hill just outside Amlwch called the Parys Mountain. It was the discovery in 1768 that this mountain was virtually composed of copper which initiated not merely the rise to power and wealth of Hughes and his business associates, but also a brief revolution in the industry itself. During the first half of the eighteenth century the trades of mining, smelting and manufacturing copper (and also brass, of which copper is a main component) had undergone a steady expansion. In the late sixteenth and seventeenth centuries copper mining and manu facturing had been monopoly industries carried on by the Mines Royal and the Mineral and Battery Companies, but towards the end revealed two brown paper parcels which contain correspondence of Michael Hughes between 1800 and 1825, with a few earlier documents. -
Removal of Iron from Dyffryn Adda, Parys Mountain, N. Wales, UK Using Sono-Electrochemistry (Electrolysis with Assisted Power Ultrasound)
Lappeenranta, Finland Mine Water and Circular Economy IMWA 2017 Removal of Iron from Dyffryn Adda, Parys Mountain, N. Wales, UK using Sono-electrochemistry (Electrolysis with assisted Power Ultrasound) Sarah A Morgan1, Zoe N Matthews1, Philip G Morgan1 and Peter Stanley2 1KP2M Ltd., C10 Ashmount Business Park, Swansea, SA6 8QR, UK [email protected] 2Natural Resources Wales, TŶ Cambria, Newport Rd., Cardiff, CF24 0TP, UK [email protected] Abstract The Dyffryn Adda from Parys Mountain, N. Wales is one of the most polluting mine waters in the UK releasing c. 10 tonnes of copper per annum and 24 tonnes of zinc per annum into the Irish Sea. The Metal Mines Strategy for Wales has ranked it first. An acid, iron rich mine water visible by its ochreous staining along 3 km of the Afon Goch Amlwch to its coastal discharge at Porth Offeiriad (Priest Port) has a negative impact on both river and coastal water quality and local businesses and communities. Several investigations using Active, Passive and Hybrid treatment processes employing conventional treatment technologies as well as Pump to sea have been considered, however successful treatment has not proven to be cost beneficial to date. This study shows that sono-electrochemical treatment (combined electrolysis and power ultrasound) to produce magnesium hydroxide can raise the pH of the water, precipitate iron as insoluble iron hydroxide [Fe(OH)2] and has the potential to preferentially precipitate other metals in their stable hydroxide forms. Extrapolating the laboratory results and methods to full scale treatment (12 l sec-1 flow rate) indicates that it is a viable Active treatment process compared to other treatment options being considered and can aid failing water bodies achieve compliance with the EU Water Framework Directive. -
Prehistoric Mining at the Great Orme
PREHISTORIC MINING AT THE GREAT ORME Criteria for the identification of early mining C. Andrew Lewis M.Phil University of Wales – Bangor Agricultural and Forest Sciences September 1996 What then? Shall we sit idly down and say "The night hath come; it is no longer day"? The night hath not yet come; we are not quite Cut off from labour by the failing light; Something remains for us to do or dare . And as the evening twilight fades away, The sky is filled with stars, invisible by day. H.W.Longfellow SUMMARY This study describes and discusses the criteria for the identification of prehistoric or early mining on the Great Orme. Particular emphasis is placed on the geological conditions of the ore deposit and how this has governed the scale and morphology of the extensive workings now recognised at the site. This was achieved by underground and surface surveys of the workings together with precise recording of geological formations through the same areas. The principal routes through the 18-19th century workings were surveyed, concentrating on localities where evidence for early phases of mining existed. Geological recording took the form of logging individual rock strata and associated mineralisation from surface exposures to the deepest sections of the mine where early mining could be identified. The artefacts and features of the prehistoric workings were also investigated, by a review of all existing historical and archaeological documentation relating to the site supplemented by evidence obtained directly through this study. From this it was possible to distinguish differences in mining artefacts and features between the prehistoric period and the 18-19th centuries. -
Abandoned Mines and the Water Environment
Abandoned mines and the water environment Science project SC030136-41 Product code: SCHO0508BNZS-E-P The Environment Agency is the leading public body protecting and improving the environment in England and Wales. It’s our job to make sure that air, land and water are looked after by everyone in today’s society, so that tomorrow’s generations inherit a cleaner, healthier world. Our work includes tackling flooding and pollution incidents, reducing industry’s impacts on the environment, cleaning up rivers, coastal waters and contaminated land, and improving wildlife habitats. This report is the result of research commissioned and funded by the Environment Agency’s Science Programme. Published by: Author(s): Environment Agency, Rio House, Waterside Drive, Dave Johnston Aztec West, Almondsbury, Bristol, BS32 4UD Hugh Potter Tel: 01454 624400 Fax: 01454 624409 Ceri Jones www.environment-agency.gov.uk Stuart Rolley Ian Watson ISBN: 978-1-84432-894-9 Jim Pritchard © Environment Agency – August 2008 Dissemination Status: Released to all regions All rights reserved. This document may be reproduced Publicly available with prior permission of the Environment Agency. Keywords: The views and statements expressed in this report are Minewater, abandoned mine, Coal Authority, Water those of the author alone. The views or statements Framework Directive, non-coal mine expressed in this publication do not necessarily represent the views of the Environment Agency and the Environment Agency’s Project Manager: Environment Agency cannot accept any responsibility for Dave Johnston –Ty Cambria, Cardiff such views or statements. Collaborator(s): This report is printed on Cyclus Print, a 100% recycled Coal Authority stock, which is 100% post consumer waste and is totally Scottish Environment Protection Agency chlorine free. -
United Kingdom Minerals Yearbook 2005 British Geological Survey United Kingdom Minerals Yearbook 2005
United Kingdom Minerals Yearbook 2005 British Geological Survey United Kingdom Minerals Yearbook 2005 Statistical data to 2004 By L E Taylor, T J Brown, P A J Lusty, K Hitchen, T B Colman and D E Highley Keyworth, Nottingham: British Geological Survey 2006 BRITISH GEOLOGICAL SURVEY © NERC 2006 All rights reserved The full range of Survey publications is available from the Copyright in materials derived from the British Geological BGS Sales Desk at Nottingham, Edinburgh and London; Survey’s work is owned by the Natural Environment see contact details below or shop online at Research Council (NERC) and/or the authority that www.geologyshop.com commissioned the work. You may not copy or adapt this publication without first obtaining permission. Contact the The London Information Office also maintains a reference BGS Intellectual Property Rights Section, British Geological collection of BGS publications including maps for consultation. Survey, Keyworth, e-mail [email protected]. The Survey publishes an annual catalogue of its maps and You may quote extracts of a reasonable length without other publications; this catalogue is available from any prior permission, provided a full acknowledgement is given of the BGS Sales Desks. of the source of the extract. The British Geological Survey carries out the geological survey of Great Britain and Northern Ireland (the latter as All communications regarding the content of this an agency service for the government of Northern Ireland), publication should be addressed to the Programme and of the surrounding continental shelf, as well as its Manager, Economic Minerals, British Geological Survey, basic research projects. It also undertakes programmes of Keyworth, Nottingham NG12 5GG British technical aid in geology in developing countries as ຜ 0115 936 3493 Fax 0115 936 3520 arranged by the Department for International Development e-mail: [email protected] and other agencies. -
Tender Instructions and Information September 2010
Tender Instructions and Information September 2010 Published by Menter Mon On behalf of the Amlwch Industrial Heritage Trust CONTENTS Page Number A. Project Background (1-5) 2 B. Budget (6) 4 C. Objectives for the Interpretation (7-16) 4 D. Audience Research (17-21) 8 E. Interpretation Requirements (22-31) 9 F. Selection Process and Deadlines (32-43) 10 ANNEX ANNEX A: Pricing Schedule ANNEX B: References ANNEX C: Tender Submission Receipt 1 Invitation to Tender Brief to Consultants For: Copper Bin Interpretation for Copper Kingdom Project A Project Background 1. The project is to design and produce an interpretive experience to tell the story of Amlwch’s Copper Mining heritage and promote the internationally significant archaeology of Parys Mountain. This is to take the form of an exhibition covering the social history of Amlwch and working life in the mines, the prehistoric and industrial archaeology of the mountain and the unique geological and environmental nature of the mines. 2. Housing this exhibition is to be the main function of the Copper Bin, a historic building in Porth Amlwch being renovated for the purpose under the Anglesey Three Towns Physical Regeneration scheme and funding from Welsh Assembly Government. The interpretation to be housed inside is being commissioned by the Amlwch Industrial Heritage Trust. The Amlwch Industrial Heritage Trust (AIHT) is a registered charity formed in 1996. Its goals are the preservation of the area’s industrial heritage, the promotion of research into the area’s history and geology and the economic regeneration of the area through tourist development of these resources. -
Mineral Reconnaissance Programme Report
Natural Environment Research Council Institute of Geological Sciences Mineral Reconnaissance Programme Report -4 A report prepared for the Department of Industry This report relates to work carried out by the Institute of Geological Sciences on behalf of the Department of Industry. The information contained herein must not be published without reference to the Director, Institute of Geological Sciences D. Ostle Programme Manager Institute of Geological Sciences Keyworth, Nottingham NG12 5GG No. 51 A reconnaissance geochemical survey of c Anglesey INSTITUTE OF GEOLOGICAL SCIENCES 8 Natural Environment Research Council 3 1 Mineral Reconnaissance Programme 1 Report NO. 51 A reconnaissance geochemical survey of Anglesey Geochemistry I D. C. Cooper, BSc, PhD Geology and mineralisation I M. J. C. Nutt, BSc, PhD Mineralogy D. J. Morgan, BSc, PhD I 8 0 Crown copyright 7 982 8 London 1982 A report prepared for the Department of industry I Mineral Reconnaissance Programme Reports 38 Geophysical evidence for a concealed eastern extension of the Tanygrisiau microgranite and its 10 Geophysical surveys around Talnotry mine, possible relationship to mineralisation Kirkcudbrightshire, Scotland 39 Copper-bearing intrusive rooks at Cairngarroch Bay, 11 A study of the space form of the Cornubian granite south-west Scotland batholith and its application to detailed gravity 40 Stratabound barium-zinc mineralisation in Dalradian surveys in Cornwall schist near Aberfeldy, Scotland: Final report 12 Mineral investigations in the Teign Valley, Devon. 41 Metalliferous -
Selenium and Other Trace Element Mobility in Waste Products and Weathered Sediments at Parys Mountain Copper Mine, Anglesey, UK
Article Selenium and Other Trace Element Mobility in Waste Products and Weathered Sediments at Parys Mountain Copper Mine, Anglesey, UK Liam A. Bullock 1,*, John Parnell 1, Magali Perez 2, Joerg Feldmann 2 and Joseph G. Armstrong 1 1 Department of Geology and Petroleum Geology, Meston Building, University of Aberdeen, King’s College, Aberdeen AB24 3UE, UK; [email protected] (J.P.); [email protected] (J.G.A.) 2 Trace Element Speciation Laboratory (TESLA), Department of Chemistry, Meston Building, University of Aberdeen, King’s College, Aberdeen AB24 3UE, UK; [email protected] (M.P.); [email protected] (J.F.) * Correspondence: [email protected]; Tel.: +44-7789-585959 Received: 16 October 2017; Accepted: 20 November 2017; Published: 22 November 2017 Abstract: The Parys Mountain copper mining district (Anglesey, North Wales) hosts exposed pyritic bedrock, solid mine waste spoil heaps, and acid drainage (ochre sediment) deposits. Both natural and waste deposits show elevated trace element concentrations, including selenium (Se), at abundances of both economic and environmental consideration. Elevated concentrations of semi- metals such as Se in waste smelts highlight the potential for economic reserves in this and similar base metal mining sites. Selenium is sourced from the pyritic bedrock and concentrations are retained in red weathering smelt soils, but lost in bedrock-weathered soils and clays. Selenium correlates with Te, Au, Bi, Cd, Hg, Pb, S, and Sb across bedrock and weathered deposits. Man-made mine waste deposits show enrichment of As, Bi, Cu, Sb, and Te, with Fe oxide-rich smelt materials containing high Pb, up to 1.5 wt %, and Au contents, up to 1.2 ppm. -
12 Pen-Y-Sarn Fawr Estate, Penysarn, Anglesey LL69 9BX
12 Pen-Y-Sarn Fawr Estate, Penysarn, Anglesey LL69 9BX ● £159,950 If you’d like a bungalow in a rural village setting not too far from the coast, this is your chance! . Spacious Detached Bungalow . LPG Central Heating System . 3 Bedrooms & Bathroom . Located Near An AONB & Coast . Lounge & Sizeable Kitchen/Diner . Gardens, Off Road Parking & Garage . Conservatory With Utility Provision . Advantage Of No Onward Chain . uPVC Double Glazing Throughout . Viewing Highly Recommended Cy merwy d pob gof al wrth baratoi’r many lion hy n, ond eu diben y w rhoi arweiniad Ev ery care has been taken with the preparation of these particulars but they are f or cyff redinol y n unig, ac ni ellir gwarantu eu bod y n f anwl gy wir. Cofiwch ofy n os bydd general guidance only and complete accuracy cannot be guaranteed. If there is any unrhy w bwy nt sy ’n neilltuol o bwy sig, neu dy lid ceisio gwiriad proff esiynol. point which is of particular importance please ask or prof essional v erification should Brasamcan y w’r holl ddimensiy nau. Nid y w cyf eiriad at ddarnau gosod a gosodiadau be sought. All dimensions are approximate. The mention of any f ixtures f ittings &/or a/neu gyf arpar y n goly gu eu bod mewn cyf lwr gweithredol eff eithlon. Darperir appliances does not imply they are in f ull eff icient working order. Photographs are ffotograff au er gwy bodaeth gyff redinol, ac ni ellir casglu bod unrhy w eitem a prov ided f or general inf ormation and it cannot be inf erred that any item shown is ddangosir y n gy nwysedig y n y pris gwerthu.