Table of Contents
Total Page:16
File Type:pdf, Size:1020Kb
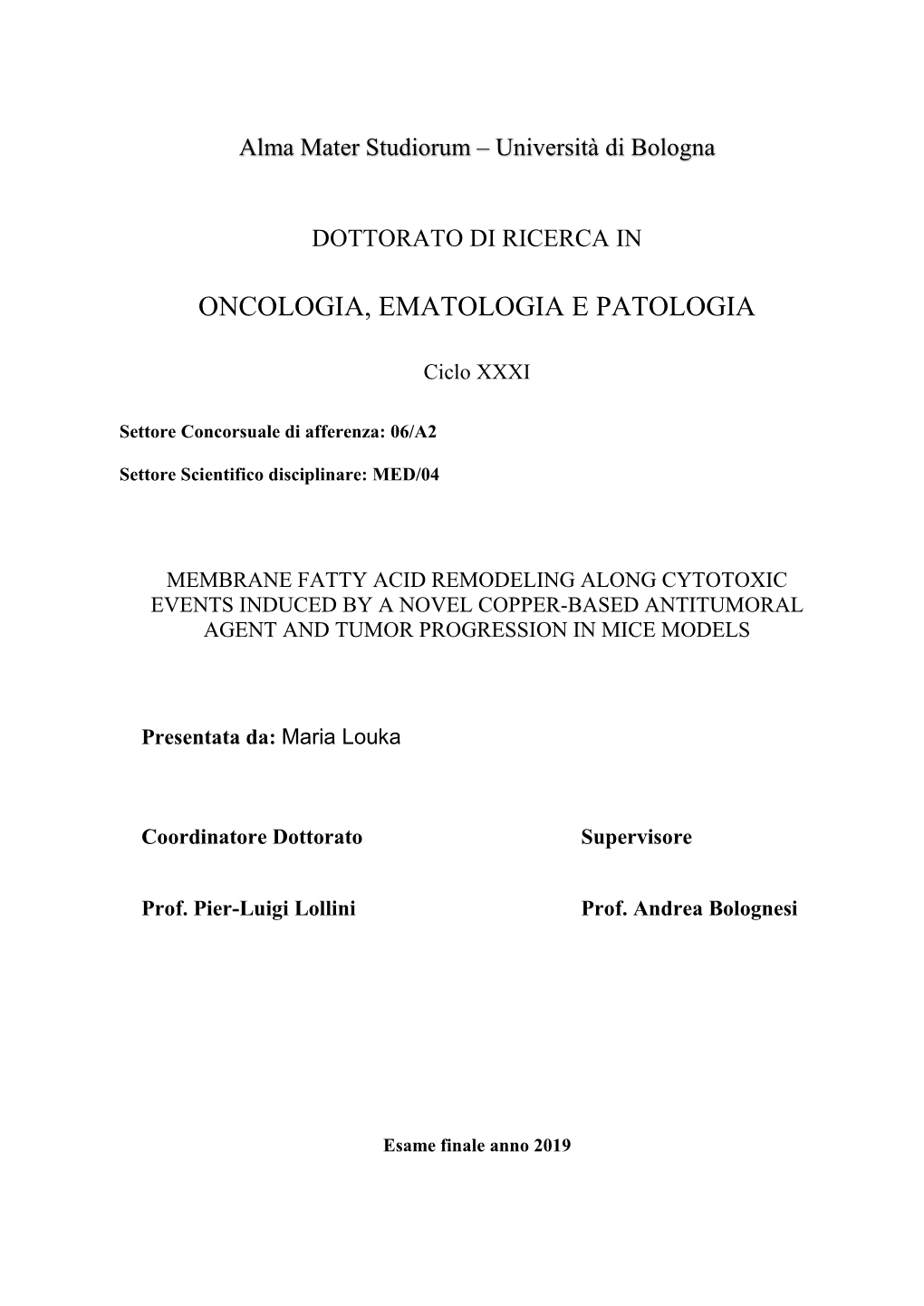
Load more
Recommended publications
-
Study on Synthesis, Characterization and Antiproliferative Activity of Novel Diisopropylphenyl Esters of Selected Fatty Acids
Journal of Oleo Science Copyright ©2016 by Japan Oil Chemists’ Society J-STAGE Advance Publication date : December 11, 2015 doi : 10.5650/jos.ess15151 J. Oleo Sci. Study on Synthesis, Characterization and Antiproliferative Activity of Novel Diisopropylphenyl Esters of Selected Fatty Acids Yasa Sathyam Reddy1, Shiva Shanker Kaki1, Bala Bhaskara Rao2, Nishant Jain2 and Penumarthy Vijayalakshmi1,* 1 Centre for Lipid Research, CSIR-Indian Institute of Chemical Technology (CSIR-IICT), Hyderabad 500007, Telangana, INDIA 2 Centre for Chemical Biology, CSIR-Indian Institute of Chemical Technology (CSIR-IICT), Hyderabad 500007, Telangana, INDIA Abstract: The present study describes the synthesis, characterization and evaluation of antiproliferative activity of novel diisopropylphenyl esters of alpha-linolenic acid (ALA), valproic acid (VA), butyric acid (BA) and 2-ethylhexanoic acid (2-EHA). These esters were chemically synthesized by the esterification of fatty acids with 2,6-diisopropylphenol and 2,4-diisopropylphenol (propofol). The structure of new conjugates viz. propofol-(alpha-linolenic acid) (2,6P-ALA and 2,4P-ALA), propofol-valproic acid (2,6P-VA and 2,4P-VA), propofol-butyric acid (2,6P-BA and 2,4P-BA) and propofol-(2-ethylhexanoic acid) (2,6P- 2-EHA and 2,4P-2-EHA) were characterized by FT-IR, NMR (1H, 13C) and mass spectral data. The synthesized conjugates having more lipophilic character were tested for antiproliferative in vitro studies on A549, MDA-MB-231, HeLa, Mia-Pa-Ca and HePG2 cancer cell lines. All the conjugates showed specific growth inhibition on studied cancer cell lines. Among the synthesized esters, the conjugates synthesized from BA, VA and 2-EHA exhibited prominent growth inhibition against A549, HeLa, Mia-Pa-Ca and HePG2 cancer cell lines. -
YEAST SMELL LIKE WHAT THEY EAT: ANALYSIS of VOLATILE ORGANIC COMPOUNDS of Malassezia Furfur in GROWTH MEDIA SUPPLEMENTED WITH
! YEAST SMELL LIKE WHAT THEY EAT: ANALYSIS OF VOLATILE ORGANIC COMPOUNDS OF Malassezia furfur IN GROWTH MEDIA SUPPLEMENTED WITH DIFFERENT LIPIDS " THESIS PRESENTED AS PARTIAL REQUIREMENT TO OBTAIN THE TITLE AS MASTER OF SCIENCE IN CHEMISTRY Presented by: MABEL CRISTINA GONZÁLEZ MONTOYA Advisor CHIARA CARAZZONE, Ph.D. Co-advisor ADRIANA M. CELIS, Ph.D." ! CHEMISTRY DEPARTMENT UNIVERSIDAD DE LOS ANDES DECEMBER 2018 BOGOTÁ D.C. ! ! !2 CONTENT 1. ABSTRACT................................................................................................................................... 6 2. ACKNOWLEDGEMENTS ............................................................................................................7 3. INTRODUCTION TO THE GENERAL TOPIC............................................................................. 8 4. PROBLEM STATEMENT AND JUSTIFICATION OF THE RESEARCH PROJECT................... 9 5. OBJECTIVES OF THE STUDY.................................................................................................. 10 5.1. General objective............................................................................................................... 10 5.2. Specific objectives............................................................................................................. 10 6. THEORETICAL FRAMEWORK................................................................................................. 10 6.1. Microbial and fungal VOCs.............................................................................................. -
Fatty Acids - from Energy Substrates to Key Regulators of Cell Survival, Proliferation and Effector Function
Review www.cell-stress.com Fatty acids - from energy substrates to key regulators of cell survival, proliferation and effector function Danilo Cucchi1, Dolores Camacho-Muñoz2, Michelangelo Certo3, Valentina Pucino3, Anna Nicolaou2,4,* and Claudio Mauro3,5,6,* 1 Barts Cancer Institute, Queen Mary University of London, Charterhouse Square, London EC1M 6BQ, UK. 2 Laboratory for Lipidomics and Lipid Biology, Division of Pharmacy and Optometry, Faculty of Biology, Medicine and Health, School of Health sciences, The University of Manchester, Manchester Academic Health Science Centre, Oxford Road, Manchester M13 9PT, UK. 3 Institute of Inflammation and Ageing, College of Medical and Dental Sciences, University of Birmingham, Mindelsohn Way, Birming- ham B15 2WB, UK. 4 Lydia Becker Institute of Immunology and Inflammation, Faculty of Biology, Medicine and Health, The University of Manchester, Manchester Academic Health Science Centre, Oxford Road, Manchester M13 9PT, UK. 5 Institute of Cardiovascular Sciences, College of Medical and Dental Sciences, University of Birmingham, Mindelsohn Way, Birming- ham B15 2WB, UK. 6 Institute of Metabolism and Systems Research, College of Medical and Dental Sciences, University of Birmingham, Mindelsohn Way, Birmingham B15 2WB, UK. * Corresponding Authors: Anna Nicolaou, Stopford Building, Oxford Road, Manchester M13 9PT, UK; E-mail: [email protected]; Claudio Mauro, Queen Elizabeth Hospital, Mindelsohn Way, Birmingham B15 2WB, UK; E-mail: [email protected] ABSTRACT Recent advances in immunology and cancer research doi: 10.15698/cst2020.01.209 show that fatty acids, their metabolism and their sensing have a cru- Received originally: 07.10.2019 cial role in the biology of many different cell types. -
Lipidquan-R for Triglycerides in Human Serum: a Rapid, Targeted UPLC-MS/MS Method for Lipidomic Research Studies Billy Joe Molloy Waters Corporation, Wilmslow, UK
[ APPLICATION NOTE ] LipidQuan-R for Triglycerides in Human Serum: A Rapid, Targeted UPLC-MS/MS Method for Lipidomic Research Studies Billy Joe Molloy Waters Corporation, Wilmslow, UK APPLICATION BENEFITS INTRODUCTION ■■ Simultaneous, targeted UPLC-MS/MS Triglycerides (TAG’s) are lipid molecules made up of a glycerol backbone and analysis of 54+ triglycerides (687 MRM three fatty acids, connected via ester linkages. They are the most abundant transitions) in a single analytical run that lipids in human serum and are the storage form for fatty acids. Historically, is under eleven minutes triglycerides have been measured as a single combined level of all triglycerides. However, this class of compound has huge variety in terms of ■■ High throughput analysis means larger sample sets can be analyzed the triglyceride species present. This variety is due to the three different fatty acid residues that make up a triglyceride, the number of carbon atoms (NC), ■■ Use of Multiple Reaction Monitoring and the number of double bonds (DB), vary from one triglyeride to another. (MRM) gives structural information Furthermore, a given triglyeride (NC:DB) might have various structural for triglycerides detected isomers due to a different combination of fatty acids giving the same NC:DB ■■ Use of a generic LC-MS configuration combination. Here we demonstrate a high-throughput UPLC-MS/MS yields versatility for switching from research method for the semi-quantitative analysis of various triglycerides in one compound class to another human serum samples. This method is capable of measuring 54 triglyceride NC:DB combinations and in excess of 100 individual triglycerides. This application note is also part of a LipidQuan-R Method Package. -
Linking Diet to Acne Metabolomics, Inflammation, and Comedogenesis: an Update
Clinical, Cosmetic and Investigational Dermatology Dovepress open access to scientific and medical research Open Access Full Text Article REVIEW Linking diet to acne metabolomics, inflammation, and comedogenesis: an update Bodo C Melnik Abstract: Acne vulgaris, an epidemic inflammatory skin disease of adolescence, is closely Department of Dermatology, related to Western diet. Three major food classes that promote acne are: 1) hyperglycemic Environmental Medicine and Health carbohydrates, 2) milk and dairy products, 3) saturated fats including trans-fats and deficient Theory, University of Osnabrück, ω-3 polyunsaturated fatty acids (PUFAs). Diet-induced insulin/insulin-like growth factor (IGF- Germany 1)-signaling is superimposed on elevated IGF-1 levels during puberty, thereby unmasking the impact of aberrant nutrigenomics on sebaceous gland homeostasis. Western diet provides abun- dant branched-chain amino acids (BCAAs), glutamine, and palmitic acid. Insulin and IGF-1 suppress the activity of the metabolic transcription factor forkhead box O1 (FoxO1). Insulin, IGF-1, BCAAs, glutamine, and palmitate activate the nutrient-sensitive kinase mechanistic target Video abstract of rapamycin complex 1 (mTORC1), the key regulator of anabolism and lipogenesis. FoxO1 is a negative coregulator of androgen receptor, peroxisome proliferator-activated receptor-γ (PPARγ), liver X receptor-α, and sterol response element binding protein-1c (SREBP-1c), crucial transcription factors of sebaceous lipogenesis. mTORC1 stimulates the expression of PPARγ and SREBP-1c, promoting sebum production. SREBP-1c upregulates stearoyl-CoA- and ∆6-desaturase, enhancing the proportion of monounsaturated fatty acids in sebum triglycerides. Diet-mediated aberrations in sebum quantity (hyperseborrhea) and composition (dysseborrhea) promote Propionibacterium acnes overgrowth and biofilm formation with overexpression of the virulence factor triglyceride lipase increasing follicular levels of free palmitate and oleate. -
Fatty Acids, Trivial and Systematic Names
FATTY ACIDS, TRIVIAL AND SYSTEMATIC NAMES Trivial Name Systematic Name Abbreviation Formic Acid Methanoic Acid Acetic Acid Ethanoic Acid Propionic Acid Propanoic Acid Butyric Acid Butanoic Acid 4:0 Valerianic Acid Pentanoic Acid 5:0 Caproic Acid Hexanoic Acid 6:0 Enanthic Acid Heptanoic Acid 7:0 Caprylic Acid Octanoic Acid 8:0 Pelargonic Acid Nonanoic Acid 9:0 Capric Acid Decanoic Acid 10:0 Obtusilic Acid 4-Decenoic Acid 10:1(n-6) Caproleic Acid 9-Decenoic Acid 10:1(n-1) Undecylic Acid Undecanoic Acid 11:0 Lauric Acid Dodecanoic Acid 12:0 Linderic Acid 4-Dodecenoic Acid 12:1(n-8) Denticetic Acid 5-Dodecenoic Acid 12:1(n-7) Lauroleic Acid 9-Dodecenoic Acid 12:1(n-3) Tridecylic Acid Tridecanoic Acid 13:0 Myristic Acid Tetradecanoic Acid 14:0 Tsuzuic Acid 4-Tetradecenoic Acid 14:1(n-10) Physeteric Acid 5-Tetradecenoic Acid 14:1(n-9) Myristoleic Acid 9-Tetradecenoic Acid 14:1(n-5) Pentadecylic Acid Pentadecanoic Acid 15:0 Palmitic Acid Hexadecanoic Acid 16:0 Gaidic acid 2-Hexadecenoic Acid 16:1(n-14) Sapienic Acid 6-Hexadecenoic Acid 16:1(n-10) Hypogeic Acid trans-7-Hexadecenoic Acid t16:1(n-9) cis-Hypogeic Acid 7-Hexadecenoic Acid 16:1(n-9) Palmitoleic Acid 9-Hexadecenoic Acid 16:1(n-7) Palmitelaidic Acid trans-9-Hexadecenoic Acid t16:1(n-7) Palmitvaccenic Acid 11-Hexadecenoic Acid 16:1(n-5) Margaric Acid Heptadecanoic Acid 17:0 Civetic Acid 8-Heptadecenoic Acid 17:1 Stearic Acid Octadecanoic Acid 18:0 Petroselinic Acid 6-Octadecenoic Acid 18:1(n-12) Oleic Acid 9-Octadecenoic Acid 18:1(n-9) Elaidic Acid trans-9-Octadecenoic acid t18:1(n-9) -
Plasticity of Coagulase-Negative Staphylococcal Membrane Fatty Acid Composition and Implications for Responses to Antimicrobial Agents
antibiotics Article Plasticity of Coagulase-Negative Staphylococcal Membrane Fatty Acid Composition and Implications for Responses to Antimicrobial Agents Kiran B. Tiwari 1, Craig Gatto 2 and Brian J. Wilkinson 2,* 1 A-TEK Inc., 1430 Spring Hill Road, McLean, VA 22102, USA 2 School of Biological Sciences, Illinois State University, Normal, IL 61761, USA * Correspondence: [email protected]; Tel.: +1-309-438-7244 Received: 28 March 2020; Accepted: 27 April 2020; Published: 28 April 2020 Abstract: Staphylococcus aureus demonstrates considerable membrane lipid plasticity in response to different growth environments, which is of potential relevance to response and resistance to various antimicrobial agents. This information is not available for various species of coagulase-negative staphylococci, which are common skin inhabitants, can be significant human pathogens, and are resistant to multiple antibiotics. We determined the total fatty acid compositions of Staphylococcus auricularis, Staphylococcus capitis, Staphylococcus epidermidis, Staphylococcus haemolyticus, Staphylococcus hominis, Staphylococcus saprophyticus, and Staphylococcus aureus for comparison purposes. Different proportions of branched-chain and straight-chain fatty acids were observed amongst the different species. However, growth in cation-supplemented Mueller–Hinton broth significantly increased the proportion of branched-chain fatty acids, and membrane fluidities as measured by fluorescence anisotropy. Cation-supplemented Mueller–Hinton broth is used for routine determination of antimicrobial susceptibilities. Growth in serum led to significant increases in straight-chain unsaturated fatty acids in the total fatty acid profiles, and decreases in branched-chain fatty acids. This indicates preformed fatty acids can replace biosynthesized fatty acids in the glycerolipids of coagulase-negative staphylococci, and indicates that bacterial fatty acid biosynthesis system II may not be a good target for antimicrobial agents in these organisms. -
An Untargeted Lipidomic Approach for Qualitative Determination of Latent Fingermark Glycerides 1 Using UPLC‐IMS
1 An untargeted lipidomic approach for qualitative determination of latent fingermark glycerides 2 using UPLC‐IMS‐QToF‐MSE 3 A.A. Frick*, C. Weyermann* 4 École des Sciences Criminelles, Université de Lausanne, Batochime, 1015 Lausanne, Switzerland 5 E‐mail: [email protected], [email protected] 6 7 Abstract 8 More detailed fundamental information is required about latent fingermark composition in order to 9 better understand fingermark properties and their impact on detection efficiency, and the physical 10 and chemical changes that occur with time following deposition. The composition of the glyceride 11 fraction of latent fingermark lipids in particular is relatively under‐investigated due in part to their high 12 structural variability and the limitations of the analytical methods most frequently utilised to 13 investigate fingermark composition. Here, we present an ultra performance liquid chromatography‐ 14 ion mobility spectroscopy‐quadrupole time‐of‐flight mass spectrometry (UPLC‐IMS‐QToF‐MSE) 15 method to characterise glycerides in charged latent fingermarks using data‐independent acquisition. 16 Di‐ and triglycerides were identified in fingermark samples from a population of 10 donors, through a 17 combination of in silico fragmentation and monitoring for fatty acid neutral losses. 23 diglycerides and 18 85 families of triglycerides were identified, with significant diversity in chain length and unsaturation. 19 21 of the most abundant triglyceride families were found to be common to most or all donors, 20 presenting potential targets for further studies to monitor chemical and physical changes in latent 21 fingermarks over time. Differences in relative peak intensities may be indicative of inter‐ and intra‐ 22 donor variability. -
Thai Hotel a Na Na Na Umri Han Atau Mitte
THAI HOTELA NA NAUS009809538B2NA UMRI HAN ATAU MITTE (12 ) United States Patent ( 10 ) Patent No. : US 9 ,809 ,538 B2 Musa et al. (45 ) Date of Patent: Nov . 7 , 2017 ( 54 ) RENEWABLE MODIFIED NATURAL Merriam -Webster , Definition for synthesis , obtained from http :/ / COMPOUNDS www .merriam -webster . com /dictionary / synthesis on Mar . 18 , 2016 . * ( 75 ) Inventors: Osama M . Musa , Kinnelon , NJ (US ) ; Smith , Janice G . Organic Chemistry . 1st ed . New York , NY : Ezat Khosravi, Gilesgate Moor (GB ) McGraw - Hill, 2006 , Chapter 7 , pp . 221- 269. * Thompson et al . Polymer Journal 1995 , 27 , 325 - 338 . * ( 73 ) Assignee : ISP INVESTMENTS LLC , Barluenga et al. Org. Lett . 2002, 4 , 2817 - 2819 . * Wilmington , DE (US ) International Search Report , PCT /US2011 / 045208 , published on Feb . 9 , 2012 ( * ) Notice : Subject to any disclaimer, the term of this patent is extended or adjusted under 35 * cited by examiner U . S . C . 154 (b ) by 977 days . Primary Examiner — Matthew Coughlin (74 ) Attorney, Agent, or Firm — Thompson Hine LLP ; (21 ) Appl . No. : 13/ 811 , 561 William J. Davis (22 ) PCT Filed : Jul. 25, 2011 (57 ) ABSTRACT ( 86 ) PCT No .: PCT/ US2011 /045208 Provided are modified natural compounds that are produced , at least in part , from ( A ) at least one epoxidized and /or $ 371 ( c ) ( 1 ) , maleated natural fatty acid , or epoxidized and /or maleated ( 2 ) , ( 4 ) Date : Mar. 25 , 2013 natural oil, and ( B ) at least one lactam having at least one ( 87) PCT Pub . No. : W02012 /018588 hydroxyl functional group , wherein the lactam may be in a blend with one or more optional alcohol( s ) . Among other PCT Pub . -
Omega-3 Versus Omega-6 Polyunsaturated Fatty Acids in the Prevention and Treatment of Inflammatory Skin Diseases
International Journal of Molecular Sciences Review Omega-3 Versus Omega-6 Polyunsaturated Fatty Acids in the Prevention and Treatment of Inflammatory Skin Diseases Anamaria Bali´c 1 , Domagoj Vlaši´c 2, Kristina Žužul 3, Branka Marinovi´c 1 and Zrinka Bukvi´cMokos 1,* 1 Department of Dermatology and Venereology, University Hospital Centre Zagreb, School of Medicine University of Zagreb, Šalata 4, 10 000 Zagreb, Croatia; [email protected] (A.B.); [email protected] (B.M.) 2 Department of Ophtalmology and Optometry, General Hospital Dubrovnik, Ulica dr. Roka Mišeti´ca2, 20000 Dubrovnik, Croatia; [email protected] 3 School of Medicine, University of Zagreb, Šalata 3, 10000 Zagreb, Croatia; [email protected] * Correspondence: [email protected] Received: 31 December 2019; Accepted: 21 January 2020; Published: 23 January 2020 Abstract: Omega-3 (!-3) and omega-6 (!-6) polyunsaturated fatty acids (PUFAs) are nowadays desirable components of oils with special dietary and functional properties. Their therapeutic and health-promoting effects have already been established in various chronic inflammatory and autoimmune diseases through various mechanisms, including modifications in cell membrane lipid composition, gene expression, cellular metabolism, and signal transduction. The application of !-3 and !-6 PUFAs in most common skin diseases has been examined in numerous studies, but their results and conclusions were mostly opposing and inconclusive. It seems that combined !-6, gamma-linolenic acid (GLA), and -
Systematic Analysis of Selective Bactericidal Activity of Fatty Acids
Journal of Oleo Science Copyright ©2019 by Japan Oil Chemists’ Society doi : 10.5650/jos.ess18220 J. Oleo Sci. 68, (3) 291-296 (2019) NOTE Systematic Analysis of Selective Bactericidal Activity of Fatty Acids against Staphylococcus aureus with Minimum Inhibitory Concentration and Minimum Bactericidal Concentration Takamasa Watanabe, Yoshiaki Yamamoto, Maki Miura, Hiroyuki Konno, Shigekazu Yano, and Yoshimune Nonomura* Department of Biochemical Engineering, Graduate School of Science and Engineering, Yamagata University, 4-3-16 Jonan, Yonezawa 992-8510, JAPAN Abstract: Bacterial flora on the skin surface contains Staphylococcus aureus (S. aureus) and Staphylococcus epidermidis (S. epidermidis) which causes rough skin and atopic dermatitis and enhances innate immunity, respectively. In this study, minimum inhibitory concentration (MIC) was evaluated for six saturated fatty acids and two unsaturated fatty acids against S. aureus and S. epidermidis. The antimicrobial behavior in the liquid medium was categorized into three groups. The first was the selective antibacterial activity group comprising myristic acid (C14:0 fatty acid), palmitoleic acid (C16:1 fatty acid), and oleic acid (C18:1 fatty acid) and preferentially displayed antimicrobial activity for S. aureus (group 1). C16:1 fatty acid displayed high antimicrobial activity only for S. aureus. The second was the non-selective antibacterial activity group which displayed antibacterial activity for both Staphylococci (group 2). Caprylic acid (C8:0 fatty acid), capric acid (C10:0 fatty acid), and lauric acid (C12:0 fatty acid) comprised group 2. The third was the non- antibacterial activity group which did not show significant antimicrobial activity (group 3). Bactericidal activities were confirmed for C12:0 fatty acid and C16:1 fatty acid by evaluating the minimum bactericidal concentration (MBC) on the agar medium. -
Oral Mucosal Lipids Are Antibacterial Against Porphyromonas Gingivalis, Induce Ultrastructural Damage, and Alter Bacterial Lipid and Protein Compositions
International Journal of Oral Science (2013) 5, 130–140 ß 2013 WCSS. All rights reserved 1674-2818/13 www.nature.com/ijos ORIGINAL ARTICLE Oral mucosal lipids are antibacterial against Porphyromonas gingivalis, induce ultrastructural damage, and alter bacterial lipid and protein compositions Carol L Fischer1, Katherine S Walters2, David R Drake1,3, Deborah V Dawson1,4, Derek R Blanchette1, Kim A Brogden1,5 and Philip W Wertz1,6 Oral mucosal and salivary lipids exhibit potent antimicrobial activity for a variety of Gram-positive and Gram-negative bacteria; however, little is known about their spectrum of antimicrobial activity or mechanisms of action against oral bacteria. In this study, we examine the activity of two fatty acids and three sphingoid bases against Porphyromonas gingivalis, an important colonizer of the oral cavity implicated in periodontitis. Minimal inhibitory concentrations, minimal bactericidal concentrations, and kill kinetics revealed variable, but potent, activity of oral mucosal and salivary lipids against P. gingivalis, indicating that lipid structure may be an important determinant in lipid mechanisms of activity against bacteria, although specific components of bacterial membranes are also likely important. Electron micrographs showed ultrastructural damage induced by sapienic acid and phytosphingosine and confirmed disruption of the bacterial plasma membrane. This information, coupled with the association of treatment lipids with P. gingivalis lipids revealed via thin layer chromatography, suggests that the plasma membrane is a likely target of lipid antibacterial activity. Utilizing a combination of two-dimensional in-gel electrophoresis and Western blot followed by mass spectroscopy and N-terminus degradation sequencing we also show that treatment with sapienic acid induces upregulation of a set of proteins comprising a unique P.