Proinsulin Trafficking Through the Secretory Pathway
Total Page:16
File Type:pdf, Size:1020Kb
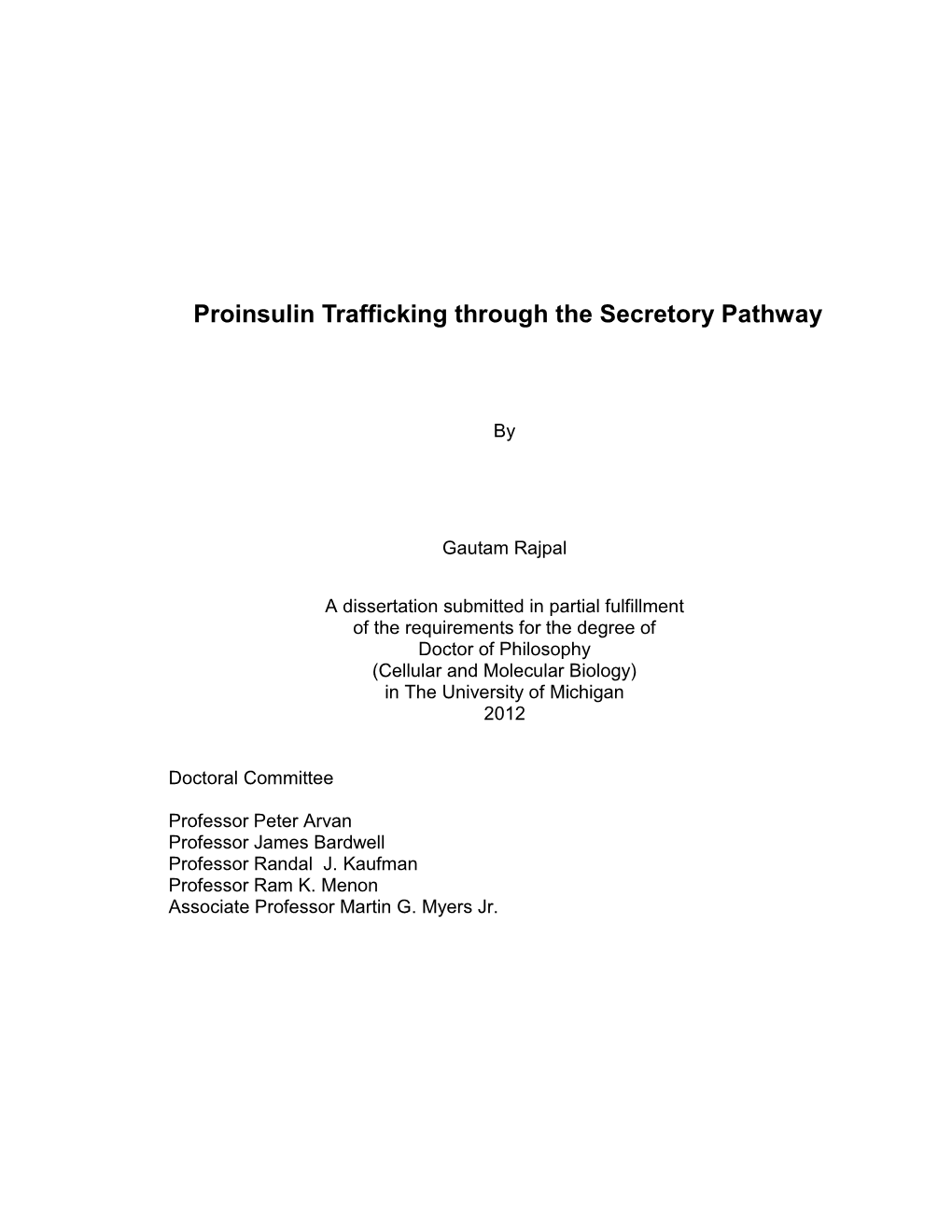
Load more
Recommended publications
-
A Computational Approach for Defining a Signature of Β-Cell Golgi Stress in Diabetes Mellitus
Page 1 of 781 Diabetes A Computational Approach for Defining a Signature of β-Cell Golgi Stress in Diabetes Mellitus Robert N. Bone1,6,7, Olufunmilola Oyebamiji2, Sayali Talware2, Sharmila Selvaraj2, Preethi Krishnan3,6, Farooq Syed1,6,7, Huanmei Wu2, Carmella Evans-Molina 1,3,4,5,6,7,8* Departments of 1Pediatrics, 3Medicine, 4Anatomy, Cell Biology & Physiology, 5Biochemistry & Molecular Biology, the 6Center for Diabetes & Metabolic Diseases, and the 7Herman B. Wells Center for Pediatric Research, Indiana University School of Medicine, Indianapolis, IN 46202; 2Department of BioHealth Informatics, Indiana University-Purdue University Indianapolis, Indianapolis, IN, 46202; 8Roudebush VA Medical Center, Indianapolis, IN 46202. *Corresponding Author(s): Carmella Evans-Molina, MD, PhD ([email protected]) Indiana University School of Medicine, 635 Barnhill Drive, MS 2031A, Indianapolis, IN 46202, Telephone: (317) 274-4145, Fax (317) 274-4107 Running Title: Golgi Stress Response in Diabetes Word Count: 4358 Number of Figures: 6 Keywords: Golgi apparatus stress, Islets, β cell, Type 1 diabetes, Type 2 diabetes 1 Diabetes Publish Ahead of Print, published online August 20, 2020 Diabetes Page 2 of 781 ABSTRACT The Golgi apparatus (GA) is an important site of insulin processing and granule maturation, but whether GA organelle dysfunction and GA stress are present in the diabetic β-cell has not been tested. We utilized an informatics-based approach to develop a transcriptional signature of β-cell GA stress using existing RNA sequencing and microarray datasets generated using human islets from donors with diabetes and islets where type 1(T1D) and type 2 diabetes (T2D) had been modeled ex vivo. To narrow our results to GA-specific genes, we applied a filter set of 1,030 genes accepted as GA associated. -
(GLP-1) in Transgenic Plants
The Open Biotechnology Journal, 2009, 3, 57-66 57 Open Access A Proficient Approach to the Production of Therapeutic Glucagon-Like Peptide-1 (GLP-1) in Transgenic Plants M. Brandsma1, X. Wang1, H. Diao2,3, S.E. Kohalmi1, A.M. Jevnikar2,3 and S. Ma1,2,3,* 1Department of Biology, University of Western Ontario, London, Ontario, N6A 5B7, Canada 2Transplantation Immunology Group, Lawson Health Research Institute, London, Ontario, N6A 4G5, Canada 3Plantigen Inc., 700 Collip Circle, London, Ontario, N6G 4X8, Canada Abstract: Glucagon-like peptide-1 (GLP-1) is a small peptide hormone with potent insulinotropic activity and represents a promising new therapeutic tool for the treatment of diabetes. Like many other therapeutic peptides, GLP-1 is commonly produced using chemical synthesis methods, but is limited by product quantity and cost. The advent of recombinant DNA technology offers the possibility of producing GLP-1 inexpensively and in vast quantities. In this study, transgenic plants were used as a recombinant expression platform for the production of GLP-1 as a large multimeric protein. A synthetic gene encoding ten sequential tandem repeats of GLP-1 sequence (GLP-1x10) was produced and introduced into tobacco plants. Transcriptional expression of the GLP1x10 gene in transgenic plants was confirmed by RT-PCR. Western blot analysis showed that the GLP-1x10 protein efficiently accumulated in transgenic plants, with an accumulation level as high as 0.15% of total soluble protein in leaves. Importantly, insulin secretion assays using a mouse pancreatic cell line (MIN6), showed that plant-derived GLP-1 in its synthetic decamer form, retained its ability to stimulate cellular insulin secretion, although with reduced efficacy. -
Primate Specific Retrotransposons, Svas, in the Evolution of Networks That Alter Brain Function
Title: Primate specific retrotransposons, SVAs, in the evolution of networks that alter brain function. Olga Vasieva1*, Sultan Cetiner1, Abigail Savage2, Gerald G. Schumann3, Vivien J Bubb2, John P Quinn2*, 1 Institute of Integrative Biology, University of Liverpool, Liverpool, L69 7ZB, U.K 2 Department of Molecular and Clinical Pharmacology, Institute of Translational Medicine, The University of Liverpool, Liverpool L69 3BX, UK 3 Division of Medical Biotechnology, Paul-Ehrlich-Institut, Langen, D-63225 Germany *. Corresponding author Olga Vasieva: Institute of Integrative Biology, Department of Comparative genomics, University of Liverpool, Liverpool, L69 7ZB, [email protected] ; Tel: (+44) 151 795 4456; FAX:(+44) 151 795 4406 John Quinn: Department of Molecular and Clinical Pharmacology, Institute of Translational Medicine, The University of Liverpool, Liverpool L69 3BX, UK, [email protected]; Tel: (+44) 151 794 5498. Key words: SVA, trans-mobilisation, behaviour, brain, evolution, psychiatric disorders 1 Abstract The hominid-specific non-LTR retrotransposon termed SINE–VNTR–Alu (SVA) is the youngest of the transposable elements in the human genome. The propagation of the most ancient SVA type A took place about 13.5 Myrs ago, and the youngest SVA types appeared in the human genome after the chimpanzee divergence. Functional enrichment analysis of genes associated with SVA insertions demonstrated their strong link to multiple ontological categories attributed to brain function and the disorders. SVA types that expanded their presence in the human genome at different stages of hominoid life history were also associated with progressively evolving behavioural features that indicated a potential impact of SVA propagation on a cognitive ability of a modern human. -
Anti-Inflammatory Role of Curcumin in LPS Treated A549 Cells at Global Proteome Level and on Mycobacterial Infection
Anti-inflammatory Role of Curcumin in LPS Treated A549 cells at Global Proteome level and on Mycobacterial infection. Suchita Singh1,+, Rakesh Arya2,3,+, Rhishikesh R Bargaje1, Mrinal Kumar Das2,4, Subia Akram2, Hossain Md. Faruquee2,5, Rajendra Kumar Behera3, Ranjan Kumar Nanda2,*, Anurag Agrawal1 1Center of Excellence for Translational Research in Asthma and Lung Disease, CSIR- Institute of Genomics and Integrative Biology, New Delhi, 110025, India. 2Translational Health Group, International Centre for Genetic Engineering and Biotechnology, New Delhi, 110067, India. 3School of Life Sciences, Sambalpur University, Jyoti Vihar, Sambalpur, Orissa, 768019, India. 4Department of Respiratory Sciences, #211, Maurice Shock Building, University of Leicester, LE1 9HN 5Department of Biotechnology and Genetic Engineering, Islamic University, Kushtia- 7003, Bangladesh. +Contributed equally for this work. S-1 70 G1 S 60 G2/M 50 40 30 % of cells 20 10 0 CURI LPSI LPSCUR Figure S1: Effect of curcumin and/or LPS treatment on A549 cell viability A549 cells were treated with curcumin (10 µM) and/or LPS or 1 µg/ml for the indicated times and after fixation were stained with propidium iodide and Annexin V-FITC. The DNA contents were determined by flow cytometry to calculate percentage of cells present in each phase of the cell cycle (G1, S and G2/M) using Flowing analysis software. S-2 Figure S2: Total proteins identified in all the three experiments and their distribution betwee curcumin and/or LPS treated conditions. The proteins showing differential expressions (log2 fold change≥2) in these experiments were presented in the venn diagram and certain number of proteins are common in all three experiments. -
Isolation and Proteomics of the Insulin Secretory Granule
H OH metabolites OH Review Isolation and Proteomics of the Insulin Secretory Granule Nicholas Norris , Belinda Yau * and Melkam Alamerew Kebede Charles Perkins Centre, School of Medical Sciences, University of Sydney, Camperdown, NSW 2006, Australia; [email protected] (N.N.); [email protected] (M.A.K.) * Correspondence: [email protected] Abstract: Insulin, a vital hormone for glucose homeostasis is produced by pancreatic beta-cells and when secreted, stimulates the uptake and storage of glucose from the blood. In the pancreas, insulin is stored in vesicles termed insulin secretory granules (ISGs). In Type 2 diabetes (T2D), defects in insulin action results in peripheral insulin resistance and beta-cell compensation, ultimately leading to dysfunctional ISG production and secretion. ISGs are functionally dynamic and many proteins present either on the membrane or in the lumen of the ISG may modulate and affect different stages of ISG trafficking and secretion. Previously, studies have identified few ISG proteins and more recently, proteomics analyses of purified ISGs have uncovered potential novel ISG proteins. This review summarizes the proteins identified in the current ISG proteomes from rat insulinoma INS-1 and INS-1E cell lines. Here, we also discuss techniques of ISG isolation and purification, its challenges and potential future directions. Keywords: insulin secretory granule; beta-cells; granule protein purification 1. Insulin Granule Biogenesis and Function Citation: Norris, N.; Yau, B.; Kebede, The insulin secretory granule (ISG) is the storage vesicle for insulin in pancreatic M.A. Isolation and Proteomics of the beta-cells. It was long treated as an inert carrier for insulin but is now appreciated as a Insulin Secretory Granule. -
WO 2019/079361 Al 25 April 2019 (25.04.2019) W 1P O PCT
(12) INTERNATIONAL APPLICATION PUBLISHED UNDER THE PATENT COOPERATION TREATY (PCT) (19) World Intellectual Property Organization I International Bureau (10) International Publication Number (43) International Publication Date WO 2019/079361 Al 25 April 2019 (25.04.2019) W 1P O PCT (51) International Patent Classification: CA, CH, CL, CN, CO, CR, CU, CZ, DE, DJ, DK, DM, DO, C12Q 1/68 (2018.01) A61P 31/18 (2006.01) DZ, EC, EE, EG, ES, FI, GB, GD, GE, GH, GM, GT, HN, C12Q 1/70 (2006.01) HR, HU, ID, IL, IN, IR, IS, JO, JP, KE, KG, KH, KN, KP, KR, KW, KZ, LA, LC, LK, LR, LS, LU, LY, MA, MD, ME, (21) International Application Number: MG, MK, MN, MW, MX, MY, MZ, NA, NG, NI, NO, NZ, PCT/US2018/056167 OM, PA, PE, PG, PH, PL, PT, QA, RO, RS, RU, RW, SA, (22) International Filing Date: SC, SD, SE, SG, SK, SL, SM, ST, SV, SY, TH, TJ, TM, TN, 16 October 2018 (16. 10.2018) TR, TT, TZ, UA, UG, US, UZ, VC, VN, ZA, ZM, ZW. (25) Filing Language: English (84) Designated States (unless otherwise indicated, for every kind of regional protection available): ARIPO (BW, GH, (26) Publication Language: English GM, KE, LR, LS, MW, MZ, NA, RW, SD, SL, ST, SZ, TZ, (30) Priority Data: UG, ZM, ZW), Eurasian (AM, AZ, BY, KG, KZ, RU, TJ, 62/573,025 16 October 2017 (16. 10.2017) US TM), European (AL, AT, BE, BG, CH, CY, CZ, DE, DK, EE, ES, FI, FR, GB, GR, HR, HU, ΓΕ , IS, IT, LT, LU, LV, (71) Applicant: MASSACHUSETTS INSTITUTE OF MC, MK, MT, NL, NO, PL, PT, RO, RS, SE, SI, SK, SM, TECHNOLOGY [US/US]; 77 Massachusetts Avenue, TR), OAPI (BF, BJ, CF, CG, CI, CM, GA, GN, GQ, GW, Cambridge, Massachusetts 02139 (US). -
Proinsulin Secretion Is a Persistent Feature of Type 1 Diabetes
258 Diabetes Care Volume 42, February 2019 Proinsulin Secretion Is a Emily K. Sims,1,2 Henry T. Bahnson,3 Julius Nyalwidhe,4 Leena Haataja,5 Persistent Feature of Type 1 Asa K. Davis,3 Cate Speake,3 Linda A. DiMeglio,1,2 Janice Blum,6 Diabetes Margaret A. Morris,7 Raghavendra G. Mirmira,1,2,8,9,10 7 10,11 Diabetes Care 2019;42:258–264 | https://doi.org/10.2337/dc17-2625 Jerry Nadler, Teresa L. Mastracci, Santica Marcovina,12 Wei-Jun Qian,13 Lian Yi,13 Adam C. Swensen,13 Michele Yip-Schneider,14 C. Max Schmidt,14 Robert V. Considine,9 Peter Arvan,5 Carla J. Greenbaum,3 Carmella Evans-Molina,2,8,9,10,15 and the T1D Exchange Residual C-peptide Study Group* OBJECTIVE Abnormally elevated proinsulin secretion has been reported in type 2 and early type 1 diabetes when significant C-peptide is present. We questioned whether individuals with long-standing type 1 diabetes and low or absent C-peptide secretory capacity retained the ability to make proinsulin. RESEARCH DESIGN AND METHODS C-peptide and proinsulin were measured in fasting and stimulated sera from 319 subjects with long-standing type 1 diabetes (‡3 years) and 12 control subjects without diabetes. We considered three categories of stimulated C-peptide: 1) 1Department of Pediatrics, Indiana University ‡ 2 School of Medicine, Indianapolis, IN C-peptide positive, with high stimulated values 0.2 nmol/L; ) C-peptide posi- 2 tive, with low stimulated values ‡0.017 but <0.2 nmol/L; and 3)C-peptide Center for Diabetes and Metabolic Diseases, Indiana University School of Medicine, Indian- <0.017 nmol/L. -
Ectopic Expression of Glucagon-Like Peptide 1 for Gene Therapy of Type II Diabetes
Gene Therapy (2007) 14, 38–48 & 2007 Nature Publishing Group All rights reserved 0969-7128/07 $30.00 www.nature.com/gt ORIGINAL ARTICLE Ectopic expression of glucagon-like peptide 1 for gene therapy of type II diabetes GB Parsons, DW Souza, H Wu, D Yu, SG Wadsworth, RJ Gregory and D Armentano Department of Molecular Biology, Genzyme Corporation, Framingham, MA, USA Glucagon-like peptide 1 (GLP-1) is a promising candidate for lowered both the fasting and random-fed hyperglycemia the treatment of type II diabetes. However, the short in vivo present in these animals. Because the insulinotropic actions half-life of GLP-1 has made peptide-based treatments of GLP-1 are glucose dependent, no evidence of hypogly- challenging. Gene therapy aimed at achieving continuous cemia was observed. Improved glucose homeostasis was GLP-1 expression presents one way to circumvent the rapid demonstrated by improvements in %HbA1c (glycated he- turnover of GLP-1. We have created a GLP-1 minigene that moglobin) and in glucose tolerance tests. GLP-1-treated can direct the secretion of active GLP-1 (amino acids 7–37). animals had higher circulating insulin levels and increased Plasmid and adenoviral expression vectors encoding the 31- insulin immunostaining of pancreatic sections. GLP-1-treated amino-acid peptide linked to leader sequences required for ZDF rats showed diminished food intake and, in the first few secretion of GLP-1 yielded sustained levels of active GLP-1 weeks following vector administration, a diminished weight that were significantly greater than endogenous levels. gain. These results demonstrate the feasibility of gene Systemic administration of expression vectors to animals therapy for type II diabetes using GLP-1 expression vectors. -
Supplementary Table 8. Cpcp PPI Network Details for Significantly Changed Proteins, As Identified in 3.2, Underlying Each of the Five Functional Domains
Supplementary Table 8. cPCP PPI network details for significantly changed proteins, as identified in 3.2, underlying each of the five functional domains. The network nodes represent each significant protein, followed by the list of interactors. Note that identifiers were converted to gene names to facilitate PPI database queries. Functional Domain Node Interactors Development and Park7 Rack1 differentiation Kcnma1 Atp6v1a Ywhae Ywhaz Pgls Hsd3b7 Development and Prdx6 Ncoa3 differentiation Pla2g4a Sufu Ncf2 Gstp1 Grin2b Ywhae Pgls Hsd3b7 Development and Atp1a2 Kcnma1 differentiation Vamp2 Development and Cntn1 Prnp differentiation Ywhaz Clstn1 Dlg4 App Ywhae Ywhab Development and Rac1 Pak1 differentiation Cdc42 Rhoa Dlg4 Ctnnb1 Mapk9 Mapk8 Pik3cb Sod1 Rrad Epb41l2 Nono Ltbp1 Evi5 Rbm39 Aplp2 Smurf2 Grin1 Grin2b Xiap Chn2 Cav1 Cybb Pgls Ywhae Development and Hbb-b1 Atp5b differentiation Hba Kcnma1 Got1 Aldoa Ywhaz Pgls Hsd3b4 Hsd3b7 Ywhae Development and Myh6 Mybpc3 differentiation Prkce Ywhae Development and Amph Capn2 differentiation Ap2a2 Dnm1 Dnm3 Dnm2 Atp6v1a Ywhab Development and Dnm3 Bin1 differentiation Amph Pacsin1 Grb2 Ywhae Bsn Development and Eef2 Ywhaz differentiation Rpgrip1l Atp6v1a Nphp1 Iqcb1 Ezh2 Ywhae Ywhab Pgls Hsd3b7 Hsd3b4 Development and Gnai1 Dlg4 differentiation Development and Gnao1 Dlg4 differentiation Vamp2 App Ywhae Ywhab Development and Psmd3 Rpgrip1l differentiation Psmd4 Hmga2 Development and Thy1 Syp differentiation Atp6v1a App Ywhae Ywhaz Ywhab Hsd3b7 Hsd3b4 Development and Tubb2a Ywhaz differentiation Nphp4 -
Signaling Peptides in Plants
lopmen ve ta e l B D io & l l o l g e y C Cell & Developmental Biology Ghorbani, et al., Cell Dev Biol 2014, 3:2 ISSN: 2168-9296 DOI: 10.4172/2168-9296.1000141 Review Article Open Access Signaling Peptides in Plants Sarieh Ghorbani1,2, Ana Fernandez1,2, Pierre Hilson3,4 and Tom Beeckman1,2* 1Department of Plant Systems Biology, VIB, B-9052 Ghent, Belgium 2Department of Plant Biotechnology and Bioinformatics, Ghent University, B-9052 Ghent, Belgium 3INRA, UMR1318, Institut Jean-Pierre Bourgin, RD10, F-78000 Versailles, France 4AgroParisTech, Institut Jean-Pierre Bourgin, RD10, F-78000 Versailles, France *Corresponding author: Tom Beeckman, Department of Plant Systems Biology, VIB, B-9052 Ghent, Belgium, Tel: 32-0-93313830; E-mail: [email protected] Rec date: May 03, 2014; Acc date: Jun 16, 2014; Pub date: Jun 18, 2014 Copyright: © 2014 Ghorbani S, et al. This is an open-access article distributed under the terms of the Creative Commons Attribution License, which permits unrestricted use, distribution, and reproduction in any medium, provided the original author and source are credited. Abstract In multicellular organisms, growth and development need to be precisely coordinated and are strongly relying on positional information. Positional control is achieved through exchanges of molecular messages between cells and tissues by means of cell-to-cell communication mechanisms. Especially in plants, accurate and well-controlled cell-to-cell communication networks are essential because of the complete absence of cell mobility and the presence of rigid cell walls. For many years, phytohormones were thought to be the main messengers exchanged between cells. -
Analysis of the Dystrophin Interactome
Analysis of the dystrophin interactome Dissertation In fulfillment of the requirements for the degree “Doctor rerum naturalium (Dr. rer. nat.)” integrated in the International Graduate School for Myology MyoGrad in the Department for Biology, Chemistry and Pharmacy at the Freie Universität Berlin in Cotutelle Agreement with the Ecole Doctorale 515 “Complexité du Vivant” at the Université Pierre et Marie Curie Paris Submitted by Matthew Thorley born in Scunthorpe, United Kingdom Berlin, 2016 Supervisor: Simone Spuler Second examiner: Sigmar Stricker Date of defense: 7th December 2016 Dedicated to My mother, Joy Thorley My father, David Thorley My sister, Alexandra Thorley My fiancée, Vera Sakhno-Cortesi Acknowledgements First and foremost, I would like to thank my supervisors William Duddy and Stephanie Duguez who gave me this research opportunity. Through their combined knowledge of computational and practical expertise within the field and constant availability for any and all assistance I required, have made the research possible. Their overarching support, approachability and upbeat nature throughout, while granting me freedom have made this year project very enjoyable. The additional guidance and supported offered by Matthias Selbach and his team whenever required along with a constant welcoming invitation within their lab has been greatly appreciated. I thank MyoGrad for the collaboration established between UPMC and Freie University, creating the collaboration within this research project possible, and offering research experience in both the Institute of Myology in Paris and the Max Delbruck Centre in Berlin. Vital to this process have been Gisele Bonne, Heike Pascal, Lidia Dolle and Susanne Wissler who have aided in the often complex processes that I am still not sure I fully understand. -
Glucagon-Like Peptide-1 and Its Class BG Protein–Coupled Receptors
1521-0081/68/4/954–1013$25.00 http://dx.doi.org/10.1124/pr.115.011395 PHARMACOLOGICAL REVIEWS Pharmacol Rev 68:954–1013, October 2016 Copyright © 2016 by The Author(s) This is an open access article distributed under the CC BY-NC Attribution 4.0 International license. ASSOCIATE EDITOR: RICHARD DEQUAN YE Glucagon-Like Peptide-1 and Its Class B G Protein–Coupled Receptors: A Long March to Therapeutic Successes Chris de Graaf, Dan Donnelly, Denise Wootten, Jesper Lau, Patrick M. Sexton, Laurence J. Miller, Jung-Mo Ahn, Jiayu Liao, Madeleine M. Fletcher, Dehua Yang, Alastair J. H. Brown, Caihong Zhou, Jiejie Deng, and Ming-Wei Wang Division of Medicinal Chemistry, Faculty of Sciences, Vrije Universiteit Amsterdam, Amsterdam, The Netherlands (C.d.G.); School of Biomedical Sciences, University of Leeds, Leeds, United Kingdom (D.D.); Drug Discovery Biology Theme and Department of Pharmacology, Monash Institute of Pharmaceutical Sciences, Parkville, Victoria, Australia (D.W., P.M.S., M.M.F.); Protein and Peptide Chemistry, Global Research, Novo Nordisk A/S, Måløv, Denmark (J.La.); Department of Molecular Pharmacology and Experimental Therapeutics, Mayo Clinic, Scottsdale, Arizona (L.J.M.); Department of Chemistry and Biochemistry, University of Texas at Dallas, Richardson, Texas (J.-M.A.); Department of Bioengineering, Bourns College of Engineering, University of California at Riverside, Riverside, California (J.Li.); National Center for Drug Screening and CAS Key Laboratory of Receptor Research, Shanghai Institute of Materia Medica, Chinese Academy of Sciences, Shanghai, China (D.Y., C.Z., J.D., M.-W.W.); Heptares Therapeutics, BioPark, Welwyn Garden City, United Kingdom (A.J.H.B.); and School of Pharmacy, Fudan University, Zhangjiang High-Tech Park, Shanghai, China (M.-W.W.) Downloaded from Abstract.