PROGRESS TOWARD a NOVEL MODEL SYSTEM to INVESTIGATE FUNGAL ENDOPHYTIC SUPPRESSION of HUMAN PATHOGENS in SPINACH by Justin Stewart Golday
Total Page:16
File Type:pdf, Size:1020Kb
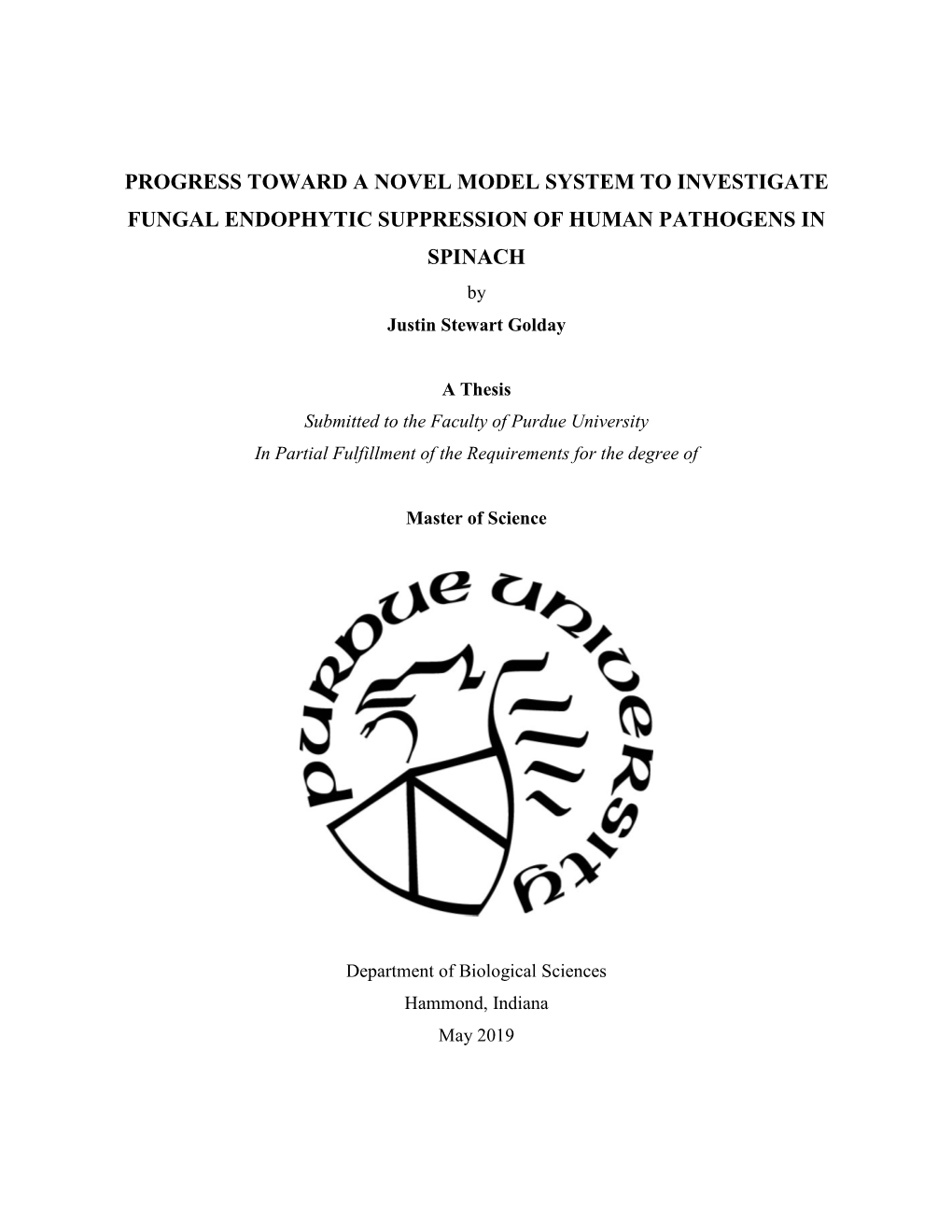
Load more
Recommended publications
-
Livro-Inpp.Pdf
GOVERNMENT OF BRAZIL President of Republic Michel Miguel Elias Temer Lulia Minister for Science, Technology, Innovation and Communications Gilberto Kassab MUSEU PARAENSE EMÍLIO GOELDI Director Nilson Gabas Júnior Research and Postgraduate Coordinator Ana Vilacy Moreira Galucio Communication and Extension Coordinator Maria Emilia Cruz Sales Coordinator of the National Research Institute of the Pantanal Maria de Lourdes Pinheiro Ruivo EDITORIAL BOARD Adriano Costa Quaresma (Instituto Nacional de Pesquisas da Amazônia) Carlos Ernesto G.Reynaud Schaefer (Universidade Federal de Viçosa) Fernando Zagury Vaz-de-Mello (Universidade Federal de Mato Grosso) Gilvan Ferreira da Silva (Embrapa Amazônia Ocidental) Spartaco Astolfi Filho (Universidade Federal do Amazonas) Victor Hugo Pereira Moutinho (Universidade Federal do Oeste Paraense) Wolfgang Johannes Junk (Max Planck Institutes) Coleção Adolpho Ducke Museu Paraense Emílio Goeldi Natural resources in wetlands: from Pantanal to Amazonia Marcos Antônio Soares Mário Augusto Gonçalves Jardim Editors Belém 2017 Editorial Project Iraneide Silva Editorial Production Iraneide Silva Angela Botelho Graphic Design and Electronic Publishing Andréa Pinheiro Photos Marcos Antônio Soares Review Iraneide Silva Marcos Antônio Soares Mário Augusto G.Jardim Print Graphic Santa Marta Dados Internacionais de Catalogação na Publicação (CIP) Natural resources in wetlands: from Pantanal to Amazonia / Marcos Antonio Soares, Mário Augusto Gonçalves Jardim. organizers. Belém : MPEG, 2017. 288 p.: il. (Coleção Adolpho Ducke) ISBN 978-85-61377-93-9 1. Natural resources – Brazil - Pantanal. 2. Amazonia. I. Soares, Marcos Antonio. II. Jardim, Mário Augusto Gonçalves. CDD 333.72098115 © Copyright por/by Museu Paraense Emílio Goeldi, 2017. Todos os direitos reservados. A reprodução não autorizada desta publicação, no todo ou em parte, constitui violação dos direitos autorais (Lei nº 9.610). -
Ass. Prof. Ahmed M. Abdel-Azeem Notes
The First International Conference of Arab Society for Fungal Conservation & Suez Canal University "Fungal Conservation in the Middle East and North of Africa" Under the auspices of; - President of Suez Canal University Prof. Mamdouh M. Ghorab - Dean of the Faculty of Science Prof. Al-Araby H. Shendy - Conference Chairman Prof. Moustafa M. Fouda - ASFC President Ass. Prof. Ahmed M. Abdel-Azeem Notes ………………………………………………………………………………………………… ………………………………………………………………………………………………… ………………………………………………………………………………………………… ………………………………………………………………………………………………… ………………………………………………………………………………………………… ………………………………………………………………………………………………… ………………………………………………………………………………………………… ………………………………………………………………………………………………… ………………………………………………………………………………………………… ………………………………………………………………………………………………… ………………………………………………………………………………………………… ………………………………………………………………………………………………… Page_2 ASFC ICFC-2016 EGYPT The First International Conference on Fungal Conservation in the Middle East and North of Africa Theme of Conference: Bioprospecting vs. Conservation Conference Booklet 18-20 October 2016 Ismailia, Egypt Page_3 ©ASFC, 2016 ©Microbial Biosystems Journal (MBJ) Print ISSN (2357-0326) II Online ISSN (2357-0334) NON-COMMERCIAL REPRODUCTION Information in this booklet has been produced with the intent that it be readily available for personal and public non-commercial use and may be reproduced, in part or in whole and by any means, without charge or further permission from Arab Society for Fungal Conservation. We ask only that: - Users exercise due diligence in ensuring -
Kavaka Title Curve-44.Cdr
VOL 44 2015 MYCOLOGICAL SOCIETY OF INDIA President PROF. B. N. JOHRI Past President PROF. T. SATYANARAYANA Vice President DR. M.V. DESHPANDE Secretary PROF. N. RAAMAN Treasurer PROF. M. SUDHAKARA REDDY Editor PROF. N.S. ATRI Editorial Board PROF. NILS HALLEMBERG, PROF. URMAS KOLJALG, PROF. B.P.R. VITTAL, PROF. ASHOK CHAVAN, PROF. S. MOHAN, KARUPPAYIL, PROF. M. CHANDRASEKARAN, PROF. K. MANJUNATH, DR. S.K. DESHMUKH, DR. R.C. UPADHYAY, PROF. SARITA W. NAZARETH, DR. M.V. DESHPANDE, DR. MUNRUCHI KAUR Members of Council PROF. N.K. DUBEY, DR. SAJAL SAJU DEO, DR. RUPAM KAPOOR, PROF. YASHPAL SHARMA, DR. AVNEET PAL SINGH, DR. SANJAY K. SINGH, DR. CHINTHALA PARAMAGEETHAM, DR. K.B. PURUSHOTHAMA, DR. K. SAMBANDAN, DR. SATISH KUMAR VERMA The Mycological Society of India was founded in January 1973 with a view to bring together the mycologists of the country and with the broad objective of promoting the development of Mycology in India in all its aspects and in the widest perspective. Memebership is open to all interested in mycology. The Life Member subscription is Rs. 3000+50/- in India and £100 or US$ 200 for those in abroad. The annual member subscription is Rs. 500+50/- in India and £20 or US $ 40 for those in abroad. Subscriptions are to be sent to the Treasurer,Prof. M. Sudhakara Reddy, Department of Biotechnology, Thaper University, Patiala-147004, Punjab, India (Email: [email protected] ). All general correspondence should be addressed toProf. N.Raaman, Secretary, MSI, C.A.S. in Botany, University of Madras, Guindy Campus, Chennai-600 025, India(Email: [email protected] ). -
Stemphylium Revisited
available online at www.studiesinmycology.org STUDIES IN MYCOLOGY 87: 77–103 (2017). Stemphylium revisited J.H.C. Woudenberg1, B. Hanse2, G.C.M. van Leeuwen3, J.Z. Groenewald1, and P.W. Crous1,4,5* 1Westerdijk Fungal Biodiversity Institute, Uppsalalaan 8, 3584 CT Utrecht, The Netherlands; 2IRS, P.O. Box 32, 4600 AA Bergen op Zoom, The Netherlands; 3National Plant Protection Organization (NPPO-NL), P.O. Box 9102, 6700 HC, Wageningen, The Netherlands; 4Wageningen University, Laboratory of Phytopathology, Droevendaalsesteeg 1, 6708 PB Wageningen, The Netherlands; 5Department of Microbiology and Plant Pathology, Forestry and Agricultural Biotechnology Institute (FABI), University of Pretoria, Pretoria 0002, South Africa *Correspondence: P.W. Crous, [email protected] Abstract: In 2007 a new Stemphylium leaf spot disease of Beta vulgaris (sugar beet) spread through the Netherlands. Attempts to identify this destructive Stemphylium sp. in sugar beet led to a phylogenetic revision of the genus. The name Stemphylium has been recommended for use over that of its sexual morph, Pleospora, which is polyphyletic. Stemphylium forms a well-defined monophyletic genus in the Pleosporaceae, Pleosporales (Dothideomycetes), but lacks an up-to-date phylogeny. To address this issue, the internal transcribed spacer 1 and 2 and intervening 5.8S nr DNA (ITS) of all available Stemphylium and Pleospora isolates from the CBS culture collection of the Westerdijk Institute (N = 418), and from 23 freshly collected isolates obtained from sugar beet and related hosts, were sequenced to construct an overview phylogeny (N = 350). Based on their phylogenetic informativeness, parts of the protein-coding genes calmodulin and glyceraldehyde-3-phosphate dehydro- genase were also sequenced for a subset of isolates (N = 149). -
Endophytic Fungi of Olive Tree and Their Exploitation in the Biological
ESCUELA SUPERIOR Y TÉCNICA DE INGENIERÍA AGRARIA INGENIERÍA DE BIOSISTEMAS Endophytic fungi of olive tree and their exploitation in the biological control of olive anthracnose Doctoral Thesis Maria de Fátima Tomé Martins Director: Professora Doutora Paula Cristina Santos Baptista León 2020 ESCUELA SUPERIOR Y TÉCNICA DE INGENIERÍA AGRARIA INGENIERÍA DE BIOSISTEMAS Hongos endofíticos del olivo y su aprovechamiento para el control biológico de la antracnosis del olivo Tesis Doctoral Maria de Fátima Tomé Martins Director: Profesora Doctora Paula Cristina Santos Baptista León 2020 This research was supported by FEDER funds through the COMPETE (Operational Programme for Competitiveness Factors) and by the Foundation for Science and Technology (FCT, Portugal) within the POCI-01-0145-FEDER-031133 project and FCT/MCTES to CIMO (UIDB/00690/2020), as well as the Horizon 2020, the European Union’s Framework Programme for Research and Innovation, for financial support the project PRIMA/0002/2018. Fátima Martins also thanks the individual research grant ref. SFRH / BD / 112234/2015 award by FCT. The studies presented in this thesis were performed at the AgroBioTechnology Laboratory, at the Mountain Research Centre (CIMO), School of Agriculture Polytechnic Institute of Bragança. Às minhas filhas Acknowledgements Neste momento, é com enorme gosto e satisfação que agradeço a todos aqueles que direta ou indiretamente contribuíram para a realização e conclusão deste trabalho. Em primeiro lugar gostaria de agradecer em especial à minha orientadora. À Professora Doutora Paula Cristina dos Santos Baptista, da Escola Superior Agrária, por toda a orientação prestada durante a realização do trabalho ao nível laboratorial e escrito, bem como, por todo o conhecimento transmitido, paciência, disponibilidade e acima de tudo pela amizade e carinho demonstrados ao longo destes anos. -
Endophytes in Maize (Zea Mays) in New Zealand
Lincoln University Digital Thesis Copyright Statement The digital copy of this thesis is protected by the Copyright Act 1994 (New Zealand). This thesis may be consulted by you, provided you comply with the provisions of the Act and the following conditions of use: you will use the copy only for the purposes of research or private study you will recognise the author's right to be identified as the author of the thesis and due acknowledgement will be made to the author where appropriate you will obtain the author's permission before publishing any material from the thesis. Endophytes in Maize (Zea mays) in New Zealand A thesis submitted in partial fulfilment of the requirements for the Degree of Master of Science at Lincoln University by Jennifer Joy Brookes Lincoln University 2017 Abstract of a thesis submitted in partial fulfilment of the requirements for the Degree of Master of Science. Abstract Endophytes in Maize (Zea mays) in New Zealand by Jennifer Joy Brookes The aim of this study was to isolate fungal endophytes from maize in New Zealand (NZ) and to select endophytes with potential to reduce insect pests and/or plant diseases. Culture methods were used to isolate 322 isolates of fungi belonging to four phyla from maize (Zea mays L.) plants. Plants were sampled over two growing seasons (2014 and 2015) in two regions of NZ. Morphological and molecular (ITS rDNA sequencing) techniques were used to identify the fungi. The most common genera recovered were Fusarium, followed by Alternaria, Trichoderma, Epicoccum, Mucor, Penicillium and Cladosoprium spp. Of the Acomycota isolates, 33 genera from 6 classes were recovered. -
Metabolite Production by Species of Stemphylium
Downloaded from orbit.dtu.dk on: Sep 28, 2021 Metabolite production by species of Stemphylium Olsen, Kresten Jon Kromphardt; Rossman, Amy; Andersen, Birgitte Published in: Fungal Biology Link to article, DOI: 10.1016/j.funbio.2017.12.012 Publication date: 2018 Document Version Peer reviewed version Link back to DTU Orbit Citation (APA): Olsen, K. J. K., Rossman, A., & Andersen, B. (2018). Metabolite production by species of Stemphylium. Fungal Biology, 122(2-3), 172-181. https://doi.org/10.1016/j.funbio.2017.12.012 General rights Copyright and moral rights for the publications made accessible in the public portal are retained by the authors and/or other copyright owners and it is a condition of accessing publications that users recognise and abide by the legal requirements associated with these rights. Users may download and print one copy of any publication from the public portal for the purpose of private study or research. You may not further distribute the material or use it for any profit-making activity or commercial gain You may freely distribute the URL identifying the publication in the public portal If you believe that this document breaches copyright please contact us providing details, and we will remove access to the work immediately and investigate your claim. Accepted Manuscript Metabolite production by species of Stemphylium Kresten Jon Kromphardt Olsen, Amy Rossman, Birgitte Andersen PII: S1878-6146(18)30001-1 DOI: 10.1016/j.funbio.2017.12.012 Reference: FUNBIO 885 To appear in: Fungal Biology Received Date: 14 November 2017 Revised Date: 18 December 2017 Accepted Date: 21 December 2017 Please cite this article as: Kromphardt Olsen, K.J., Rossman, A., Andersen, B., Metabolite production by species of Stemphylium, Fungal Biology (2018), doi: 10.1016/j.funbio.2017.12.012. -
A Worldwide List of Endophytic Fungi with Notes on Ecology and Diversity
Mycosphere 10(1): 798–1079 (2019) www.mycosphere.org ISSN 2077 7019 Article Doi 10.5943/mycosphere/10/1/19 A worldwide list of endophytic fungi with notes on ecology and diversity Rashmi M, Kushveer JS and Sarma VV* Fungal Biotechnology Lab, Department of Biotechnology, School of Life Sciences, Pondicherry University, Kalapet, Pondicherry 605014, Puducherry, India Rashmi M, Kushveer JS, Sarma VV 2019 – A worldwide list of endophytic fungi with notes on ecology and diversity. Mycosphere 10(1), 798–1079, Doi 10.5943/mycosphere/10/1/19 Abstract Endophytic fungi are symptomless internal inhabits of plant tissues. They are implicated in the production of antibiotic and other compounds of therapeutic importance. Ecologically they provide several benefits to plants, including protection from plant pathogens. There have been numerous studies on the biodiversity and ecology of endophytic fungi. Some taxa dominate and occur frequently when compared to others due to adaptations or capabilities to produce different primary and secondary metabolites. It is therefore of interest to examine different fungal species and major taxonomic groups to which these fungi belong for bioactive compound production. In the present paper a list of endophytes based on the available literature is reported. More than 800 genera have been reported worldwide. Dominant genera are Alternaria, Aspergillus, Colletotrichum, Fusarium, Penicillium, and Phoma. Most endophyte studies have been on angiosperms followed by gymnosperms. Among the different substrates, leaf endophytes have been studied and analyzed in more detail when compared to other parts. Most investigations are from Asian countries such as China, India, European countries such as Germany, Spain and the UK in addition to major contributions from Brazil and the USA. -
Fungal Flora of Korea
Fungal Flora of Korea Volume 1, Number 2 Ascomycota: Dothideomycetes: Pleosporales: Pleosporaceae Alternaria and Allied Genera 2015 National Institute of Biological Resources Ministry of Environment Fungal Flora of Korea Volume 1, Number 2 Ascomycota: Dothideomycetes: Pleosporales: Pleosporaceae Alternaria and Allied Genera Seung Hun Yu Chungnam National University Fungal Flora of Korea Volume 1, Number 2 Ascomycota: Dothideomycetes: Pleosporales: Pleosporaceae Alternaria and Allied Genera Copyright ⓒ 2015 by the National Institute of Biological Resources Published by the National Institute of Biological Resources Environmental Research Complex, Hwangyeong-ro 42, Seo-gu Incheon, 404-708, Republic of Korea www.nibr.go.kr All rights reserved. No part of this book may be reproduced, stored in a retrieval system, or transmitted, in any form or by any means, electronic, mechanical, photocopying, recording, or otherwise, without the prior permission of the National Institute of Biological Resources. ISBN : 9788968111259-96470 Government Publications Registration Number 11-1480592-000905-01 Printed by Junghaengsa, Inc. in Korea on acid-free paper Publisher : Kim, Sang-Bae Author : Seung Hun Yu Project Staff : Youn-Bong Ku, Ga Youn Cho, Eun-Young Lee Published on March 1, 2015 The Flora and Fauna of Korea logo was designed to represent six major target groups of the project including vertebrates, invertebrates, insects, algae, fungi, and bacteria. The book cover and the logo were designed by Jee-Yeon Koo. Preface The biological resources represent all the composition of organisms and genetic resources which possess the practical and potential values essential for human lives, and occupies a firm position in producing highly value-added products such as new breeds, new materials and new drugs as a means of boosting the national competitiveness. -
Risk Assessment of Secondary Metabolites Produced by Fungi in the Genus Stemphylium
Canadian Journal of Microbiology Risk assessment of secondary metabolites produced by fungi in the genus Stemphylium Journal: Canadian Journal of Microbiology Manuscript ID cjm-2020-0351.R1 Manuscript Type: Review Date Submitted by the 29-Oct-2020 Author: Complete List of Authors: Stricker, Sara; University of Guelph, Plant Agriculture Gossen, Bruce D.; Agriculture and Agri-Food Canada, Saskatoon Research and Development Centre; McDonald,Draft Mary Ruth; University of Guelph, Plant Agriculture Keyword: Stemphylium, toxins, allergen, Pleospora Is the invited manuscript for consideration in a Special Not applicable (regular submission) Issue? : © The Author(s) or their Institution(s) Page 1 of 19 Canadian Journal of Microbiology 1 Risk assessment of secondary metabolites 2 produced by fungi in the genus Stemphylium 3 4 Sara M. Stricker1, Bruce D. Gossen2, Mary Ruth McDonald1* 5 6 1Department of Plant Agriculture, University of Guelph, Guelph, Ontario, Canada 7 8 2Agriculture and Agri-Food Canada, Saskatoon Research Centre, Saskatoon, Saskatchewan. 9 Canada. 10 11 * [email protected] (MRM) 12 Draft 1 © The Author(s) or their Institution(s) Canadian Journal of Microbiology Page 2 of 19 13 Abstract 14 The fungal genus Stemphylium (phylum Ascomycota, teleomorph Pleospora) includes plant 15 pathogenic, endophytic, and saprophytic species with worldwide distributions. Stemphylium spp. 16 produce prodigious numbers of air-borne spores, so are a human health concern as allergens. 17 Some species also produce secondary metabolites such as glucosides, ferric chelates, aromatic 18 polyketides, and others that function as toxins that damage plants and other fungal species. Some 19 of these compounds also exhibit a low level of mammalian toxicity. -
The Endophytic Fungus Stemphylium Globuliferum – Secondary
The endophytic fungus Stemphylium globuliferum – secondary metabolites and biological activities Dissertation zur Erlangung des Doktorgrades (Dr. rer. nat.) der Mathematisch-Naturwissenschaftlichen Fakultät der Rheinischen Friedrich-Wilhelms-Universität Bonn vorgelegt von Jan-Philipp Schrör aus Moers Bonn 2017 Angefertigt mit Genehmigung der Mathematisch-Naturwissenschaftlichen Fakultät der Rheinischen Friedrich-Wilhelms-Universität Bonn 1. Referentin : Prof. Dr. G. M. König 2. Referent : Prof. Dr. W. Knöss Tag der Promotion : 02. November 2017 Vorveröffentlichungen der Dissertation / In Advance Publications of the Dissertation Teilergebnisse aus dieser Arbeit wurden mit Genehmigung der Mathematisch- Naturwissenschaftlichen Fakultät, vertreten durch die Mentorin/Betreuerin der Arbeit, in folgenden Beiträgen vorab veröffentlicht: Parts of this study have been published in advance by permission of the Mathematisch- Naturwissenschaftlichen Fakultät, represented by the supervisor of this study: Publikationen / Research Papers J. Schrör, S. Kehraus, N. Merten, G. M. König; “Stemphyloxin III and stemphylofuran, new insights into the mold fungus Stemphylium globuliferum”; 2017 , in preparation. J. Seungwon, J. Lee, J. Schrör, C. Cerella, S. Chateauvieux, B. Orlikova, K. Kim, C. Christov, M. Dicato, B. Han, G. M. König, M. Diederich; “The dialkylresorcinol stemphol induces necroptosis by interference with endoplasmic reticulum/mitochondrial calcium homeostasis”; 2017 , in preparation. H. Harms, A. Kloeckner, J. Schrör, S. Kehraus, M. Crüsemann, G.M. König, T.F.Schaeberle; “Antibiotic dialkylresorcins and derivatives from marine-derived organisms, insights in their mode of action and putative ecological role”, 2017 , in preparation. Tagungsbeiträge / Research presentations J. Schrör, P. Hufendiek, S. Kehraus, M. Gütschow, G. M. König; “Secondary metabolites from the fungus Stemphylium globuliferum and their biological activities”, 2015 , Poster presented at the Marine Fungal Natural Product Consortium, Nantes, France J. -
Fungal Endophytes: Isolation, Identification and Assessment of Bioactive Potential of Their Natural Products
FUNGAL ENDOPHYTES: ISOLATION, IDENTIFICATION AND ASSESSMENT OF BIOACTIVE POTENTIAL OF THEIR NATURAL PRODUCTS By Edson Panganayi Sibanda A Thesis by Manuscripts Submitted in Fulfilment of the Requirement of the Degree of Doctor of Philosophy (PhD) Department of Human Physiology, School of Laboratory Medicine and Medical Sciences, University of KwaZulu-Natal, Durban, South Africa Supervisors: Professor Takafira Mduluza, College of Health Sciences Professor Musa V. Mabandla, College of Health Sciences 20 October 2017 PREFACE The work contained in this thesis has not been previously submitted for a degree or diploma at any other higher education institutions to the best of my knowledge and belief. This thesis contains no material previously published or submitted for publication by another person except where due reference has been made. Signed: ………………………………………. Date: 20 October 2017 Edson Panganayi Sibanda ii DECLARATION 1: PLAGIARISM I EDSON PANGANAYI SIBANDA declare that: (i) The research reported in this dissertation, except where otherwise indicated, is my original work. (ii) This dissertation has not been submitted for any degree or examination at any other university. (iii) This dissertation does not contain other persons’ data, pictures, graphs or other information, unless specifically acknowledged as being sourced from other persons. (iv) This dissertation does not contain other persons’ writing, unless specifically acknowledged as being sourced from other researchers. Where other written sources have been quoted, then: a. their words have been re-written but the general information attributed to them has been referenced; b. where their exact words have been used, their writing has been placed inside quotation marks, and referenced. (v) Where I have reproduced a publication of which I am an author, co-author or editor, I have indicated in detail which part of the publication was written by myself alone and have fully referenced such publications.