I Synthesis of Structurally and Stereochemically Diverse Tetrahydropyran Structures Via DDQ-Mediated Oxidative Carbon‒Hydrogen Bond Activation
Total Page:16
File Type:pdf, Size:1020Kb
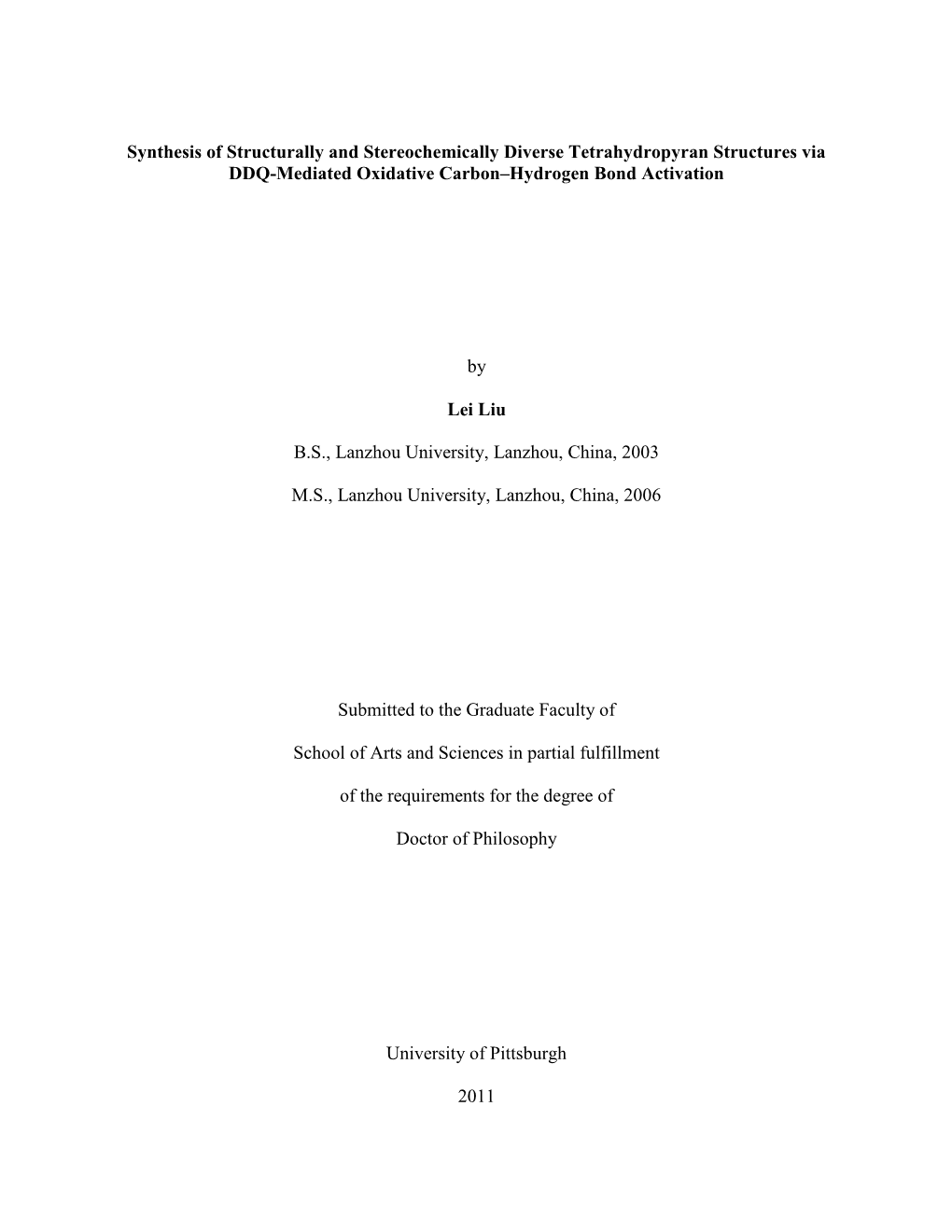
Load more
Recommended publications
-
New Methodologies for the Synthesis Of
NEW METHODOLOGIES FOR THE SYNTHESIS OF ORGANOPHOSPHORUS COMPOUNDS by MONIKA I. ANTCZAK Master of Science, 2002 Wroclaw University of Technology Wroclaw, Poland Submitted to the Graduate Faculty of the College of Science and Engineering Texas Christian University in partial fulfillment of the requirements for the degree of Doctor of Philosophy December 2008 NEW METHODOLOGIESFOR THE SYNTHESISOF ORGANOPHOSPHORUSCOMPOLNDS by MonikaI. Antczak Dissertationapproved: Maior Professor f Scienceand Engineering Copyright by Monika I. Antczak 2008 ACKNOWLEDGMENTS I wish to acknowledge Prof. Jean-Luc Montchamp for the constant interest in the progress of my work over the past five years. I also wish to thank him for his advice and intense discussions, which helped me in expanding my knowledge and understanding of organic chemistry, and in guiding me in my educational progress at Texas Christian University. His patience and support helped me overcome some difficult situation and successfully completing my Ph.D. program. I express my gratitude to my colleagues and friends, Jennifer Tellez, Dr. Laëtitia Coudray, Yamina Belabassi and Dr. Clemence Queffelec for their help, guidance and support. I also would like to thank Dr. Onofrio Annunziata, Dr. Waldek Zerda and Dr. Dale Huckaby for their guidance and interesting discussions during these years. I wish to thank: Monika Wieligor and Dr. Mauricio Quiroz for their friendship and constant support. Finally, I wish to thank my parents for their unconditional love and support. The Chemistry Department at Texas Christian University, the National Science Foundation (CHE-0242898), the National Institute of General Medical Sciences/NIH (R01 GM067610), and the Robert A. Welch Foundation (P-1666) are gratefully acknowledged for the financial support of this research. -
A Conceptual Design of Fluorous Surfactants Based on a Heterocyclic Core, Put Into Practice Through Synthesis of Fluorous 1,2,3-Triazoles Roger W
Chiang Mai J. Sci. 2009; 36(2) 247 Chiang Mai J. Sci. 2009; 36(2) : 247-257 www.science.cmu.ac.th/journal-science/josci.html Invited Paper A Conceptual Design of Fluorous Surfactants Based on a Heterocyclic Core, Put into Practice Through Synthesis of Fluorous 1,2,3-Triazoles Roger W. Read* and Xiao Bei Wang School of Chemistry, The University of New South Wales, UNSW Sydney NSW 2052, Australia. *Author for correspondence; e-mail: [email protected] ABSTRACT The concept of a design of fluorous surfactants based on a heterocyclic core molecule is introduced and illustrated through the synthesis of polyfluoroalkyl-substituted 1,2,3-triazoles. A two step, one-pot process is described in which a small range of perfluoroalkylethyl azides is prepared in situ and made to undergo Huisgen 1,3-dipolar cycloaddition with terminal alkynes. Measurement of the changes in surface tension of the library of fluorous triazoles at different concentrations in m-xylene reveals unusual behaviour in molecules with a secondary, C8 alkyl substituent. Keywords: organic synthesis, heterocycles, fluorous chemistry, surfactants, click chemistry, polyfluoroalkyl-1,2,3-triazoles. 1. INTRODUCTION Fluorous surfactants have been studied might have applications in the design of new widely, not least for their interest in drug delivery systems and, through self- combination with perfluorocarbons, as assembly, unique molecular devices. emergency blood replacements.[1] Highly Considerable literature exists in the area of fluorinated amphiphiles and colloid systems fluorous block polymers with localised are chemically stable and can carry high crystalline segments and fluorous liposomes concentrations of oxygen and carbon dioxide, and vesicles for drug delivery, but, with a few and therefore have many applications in the exceptions [8-11], most fluorous components biomedical field [2,3]. -
1-Iodo-1-Pentyne
MIAMI UNIVERSITY-THE GRADUATE SCHOOL CERTIFICATE FOR APPROVING THE DISSERTATION We hereby approve the Dissertation of Lizhi Zhu Candidate for the Degree: Doctor of Philosophy ________________________________ Robert E. Minto, Director ________________________________ John R. Grunwell, Reader ________________________________ John F. Sebastian, Reader ________________________________ Ann E. Hagerman, Reader ________________________________ Richard E. Lee, Graduate School Representative ABSTRACT INVESTIGATING THE BIOSYNTHESIS OF POLYACETYLENES: SYNTHESIS OF DEUTERATED LINOLEIC ACIDS & MECHANISM STUDIES OF DMDS ADDITION TO 1,4-ENYNES By Lizhi Zhu A wide range of polyacetylenic natural products possess antimicrobial, antitumor, and insecticidal properties. The biosyntheses of these natural products are widely distributed among fungi, algae, marine sponges, and higher plants. As details of the biosyntheses of these intriguing compounds remains scarce, it remains important to develop molecular probes and analytical methods to study polyacetylene secondary metabolism. An effective pathway to prepare selectively deuterium-labeled linoleic acids was developed. By this Pd-catalyzed method, deuterium can be easily introduced into the vinyl position providing deuterolinoleates with very high isotopic purity. This method also provides a general route for the construction of 1,4-diene derivatives with different chain lengths and 1,4-diene locations. Linoleic acid derivatives (12-d, 13-d and 16,16,17,17,18,18,18-d7) were synthesized according to this method. A stereoselective synthesis of methyl (14Z)- and (14E)-dehydrocrepenynate was achieved in five to six steps that employed Pd-catalyzed cross-coupling reactions to construct the double bonds between C14 and C15. Compared with earlier methods, the improved syntheses are more convenient (no spinning band distillations or GLC separation of diastereomers were necessary) and higher Z/E ratios were obtained. -
Gfsorganics & Fragrances
Chemicals for Flavors GFSOrganics & Fragrances GFS offers a broad range of specialty organic chemicals Specialized chemistries we as building blocks and intermediates for the manufacture of offer include: flavors and fragrances. • Alkynes Over 5,500 materials, including 1,400 chemicals from natural sources, are used for flavor • Alkynols enhancements and aroma profiles. These aroma chemicals are integral components of • Olefins the continued growth within consumer products and packaged foods. The diversity of products can be attributed to the broad spectrum of organic compounds derived from • Unsaturated Acids esters, aldehydes, lactones, alcohols and several other functional groups. • Unsaturated Esters • DIPPN and other Products GFS Chemicals manufactures a wide range of organic intermediates that have been utilized in a multitude of personal care applications. For example, we support Why GFS? several market leading companies in the manufacture and supply of alkyne based aroma chemicals. • Specialized Chemistries • Tailored Specifications As such, we understand the demanding nature of this fast changing market and are fully • From Grams to Metric Tons equipped to overcome process challenges and manufacture the novel chemical products • Responsive Technical Staff that you need, when you need them. We offer flexible custom manufacturing services to produce high purity products with the assurance of quality and full confidentiality. • Uncompromised Product Quality Our state-of-the-art manufacturing facility, located in Columbus, OH is ISO 9001:2008 certified. As a U.S. based manufacturer we have a proven record of helping you take products from development to commercialization. Our technical sales experts are readily accessible to discuss your project needs and unique product specifications. -
Chemical in Baobab (Adansonia Digitata) from Sudan
Vol. 14(21), pp. 907-914, 23 May, 2019 DOI: 10.5897/AJAR2019.13862 Article Number: 83F45A861057 ISSN: 1991-637X Copyright ©2019 African Journal of Agricultural Author(s) retain the copyright of this article http://www.academicjournals.org/AJAR Research Full Length Research Paper Identification of 1-decyne as a new volatile allele- chemical in baobab (Adansonia digitata) from Sudan Hala Sad Alla Maliek Elmadni1,2, Maryia Mishyna1,3 and Yoshiharu Fujii1,2* 1Department of International Environmental and Agricultural Science, Faculty of Agriculture, Tokyo University of Agriculture and Technology, 183-8509 Tokyo, Japan. 2Department of General Administration of technology Transfer and Extension, Federal Ministry of Agriculture and Forest, 461 Khartoum, Sudan. 3School of Food Science and Bioengineering, Zhejiang Gongshang University, Hangzhou, China. Received 3 January, 2019; Accepted 19 April, 2019 Leaf, fruit, wood, and gum of fifty-five plants collected in Sudan were evaluated by Dish pack method for their allelopathic activity through volatile chemicals using lettuce (Lactuca sativa) as a receptor plant. Several potential plants with high allelopathic activity, such as Terminalia brownie, Euphorbia hirta, Diospyros mespiliformis, Corchorus olitorius, Adansonia digitata, Hibiscus sabdariffa, were determined. Baobab (A. digitata) leaves demonstrated relatively higher inhibition (23.9 and 21.5% of hypocotyl and radicle, respectively) than most of the screened plant species. Identification of the volatile compounds using headspace gas chromatography-mass spectrometry revealed 1-decyne as the main volatile compound naturally released from dried baobab leaves. EC50 (50% growth inhibition) of radicle and hypocotyl growth of lettuce seedlings by authentic 1-decyne was determined in the headspace air using by GC-MS with Cotton Swab method at the concentration of 0.5 ng/ml. -
I LATE TRANSITION METAL-CATALYST
LATE TRANSITION METAL-CATALYST DEVELOPMENT FOR ALKYNE COUPLING REACTIONS A Dissertation Submitted to the Graduate Faculty of the North Dakota State University of Agriculture and Applied Science By Rajith Singh Manan In Partial Fulfillment of the Requirements for the Degree of DOCTOR OF PHILOSOPHY Major Department: Chemistry and Biochemistry May 2016 Fargo, North Dakota i North Dakota State University Graduate School Title LATE TRANSITION METAL-CATALYST DEVELOPMENT FOR ALKYNE COUPLING REACTIONS By Rajith Singh Manan The Supervisory Committee certifies that this disquisition complies with North Dakota State University’s regulations and meets the accepted standards for the degree of DOCTOR OF PHILOSOPHY SUPERVISORY COMMITTEE: Prof. Pinjing Zhao Chair Prof. Mukund Sibi Prof. Gregory Cook Prof. Sanku Malik Approved: June 01, 2016 Prof. Gregory Cook Date Department Chair ii ABSTRACT Alkynes are versatile and valuable synthetic precursors in a variety of organic transformations and have found tremendous applications in materials science and pharmaceutical chemistry. In recent years, there has been a tremendous growth in the development of transition- metal catalyzed organic transformations by alkynes coupling reactions such as ring constructions (carbocycles and heterocycles), hydroarylation and hydroamination. This thesis describes the development of new transition metal catalyst for alkyne coupling reactions. The results are highlighted by chemoselective coupling between internal alkynes and N-H aromatic ketimines to synthesize indenamine, -
Well-Defined Silica-Supported Molybdenum Nitride Species: Silica Grafting Triggers Alkyne Metathesis Activity
Electronic Supplementary Material (ESI) for Chemical Science This journal is © The Royal Society of Chemistry 2013 Well-defined silica-supported molybdenum nitride species: Silica grafting triggers alkyne metathesis activity. Marie Genelota, Nicolas P. Chevalb, Marta Vitorinoa, Elise Berriera, Jean-Marc Weibel,b Patrick Paleb, André Mortreuxa and Régis M. Gauvina,* a Unité de Catalyse et Chimie du Solide UCCS, CNRS UMR8181, Université Lille Nord de France, ENSCL, Cité Scientifique, Bat C7, 59652 Villeneuve d'Ascq, France Phone: +33 (0)320436754; Fax: +33(0)320436585, E-mail: [email protected] b Laboratoire de Synthèse et Réactivité Organiques, CNRS UMR7177 Institut de Chimie, Université de Strasbourg, 4 rue Blaise Pascal, 67000 Strasbourg, France. Supporting Information Table of contents: 1) General procedures S2 2) Preparation and characterization of catalyst 2 S4 3) Self-metathesis reactions S6 4) Influence of the B(C6F5)3 co-catalyst loading S7 5) Cross-metathesis reactions S9 6) Description of attempts to observe nitrile by-products S12 7) Synthesis and characterization of compound 9 S12 8) Synthesis and characterization of compound 11 S14 9) Independent synthesis of 10 and 13 S15 S1 Electronic Supplementary Material (ESI) for Chemical Science This journal is © The Royal Society of Chemistry 2013 1) General procedures: All experiments were conducted under a strict inert atmosphere using standard Schlenk and glove box techniques for the organometallic synthesis and grafting processes. Solvents were purified and dried according to standard procedures. 4-decyne and 1-phenyl-1-propyne were distilled over LiAlH4, degassed and stored in a glove-box. GC analyses were performed on a Shimadzu GC2014 equiped with a FID detector and using N2 as carrier gas. -
Platinum-Catalyzed Hydrosilylation in Polymer Chemistry
polymers Review Platinum-Catalyzed Hydrosilylation in Polymer Chemistry Ruslan Yu. Lukin 1,2,*, Aidar M. Kuchkaev 1,2, Aleksandr V. Sukhov 1,2, Giyjaz E. Bekmukhamedov 1,2 and Dmitry G. Yakhvarov 1,2,* 1 Alexander Butlerov Institute of Chemistry, Kazan Federal University, 420008 Kazan, Russia; [email protected] (A.M.K.); alex.suhoff@rambler.ru (A.V.S.); [email protected] (G.E.B.) 2 Arbuzov Institute of Organic and Physical Chemistry, FRC Kazan Scientific Center of the Russian Academy of Sciences, 420088 Kazan, Russia * Correspondence: [email protected] (R.Y.L.); [email protected] (D.G.Y.); Tel.: +7-843-2337416 (R.Y.L. & D.G.Y.); Fax: +7-843-2732253 (R.Y.L. & D.G.Y.) Received: 20 July 2020; Accepted: 15 September 2020; Published: 23 September 2020 Abstract: This paper addresses a review of platinum-based hydrosilylation catalysts. The main field of application of these catalysts is the curing of silicone polymers. Since the 1960s, this area has developed rapidly in connection with the emergence of new polymer compositions and new areas of application. Here we describe general mechanisms of the catalyst activity and the structural effects of the ligands on activity and stability of the catalysts together with the methods for their synthesis. Keywords: silicone polymers; hydrosilylation; organometallic catalysts; platinum complexes 1. Introduction The hydrosilylation reaction (also referred to as hydrosilation) is widely used in the organosilicon industry. This reaction represents the addition of silicon-hydrogen bonds (Si–H) via an unsaturated carbon–carbon double bond (C=C), carbon–oxygen, and carbon–nitrogen double bonds. -
Rollover in the Synthesis of Polycyclic Indoles, and a One-Pot Zipper-Click Reaction
Rollover in the synthesis of polycyclic indoles, and a one-pot Zipper-Click reaction A thesis submitted to the University of Manchester for the degree of Doctor of Philosophy (PhD) in the Faculty of Engineering and Physical Science 2015 Sinead Balgobin School of Chemistry ii Contents 1. C–H activation for alkyne insertion ........................................................... 1 1.1. Introduction ........................................................................................ 1 1.1.1. C–H bond activation and functionalisation .................................... 1 1.1.2. Palladium in C–H bond activation and functionalisation ............... 5 1.1.3. Rhodium in C–H bond activation and functionalisation .............. 13 1.1.4. Ruthenium in C–H bond activation and functionalisation ............ 20 1.1.5. This work ................................................................................... 30 1.2. Results and Discussion ...................................................................... 31 1.2.1. Introduction ............................................................................... 31 1.2.2. Pd-catalysed alkyne insertion ...................................................... 36 1.2.3. Rh-catalysed alkyne insertion ...................................................... 48 1.2.4. Ru-catalysed alkyne insertion ...................................................... 65 1.3. Recent literature developments .......................................................... 69 1.4. Conclusion ...................................................................................... -
(12) United States Patent (10) Patent No.: US 6,359,164 B1 Wang Et Al
USOO6359164B1 (12) United States Patent (10) Patent No.: US 6,359,164 B1 Wang et al. (45) Date of Patent: Mar. 19, 2002 (54) PROCESS FOR THE PREPARATION OF OTHER PUBLICATIONS CYCLOPROPYLACETYLENE Craig et al., Angew. Chem. Int. Ed. Engl., (1969), 8(6), 429-437. (76) Inventors: Zhe Wang, 67 Westwoods Blvd., Schoberth and Hanack, Synthesis (1972), (12), 703. Hockessin, DE (US) 19707; Joseph M. Taguchi et al., J. Am. Chem. Soc., (1974), 96(9),3010-3011. Fortunak, 19 Somerset La., Newark, Wong and Ho, Synthetic Communications, (1974), 4(1), DE (US) 19711 25-27. Notice: Subject to any disclaimer, the term of this Villieras et al., Synthesis, (1975), 458–461. patent is extended or adjusted under 35 Tsuji et al., Chemistry Letters, (1979), 481-482. Van Hijfte et al., Tetrahedron Letters, (1989), 30(28) U.S.C. 154(b) by 0 days. 3655-3656. Corey et al., Tetrahedron Letters, (1992), 33(24), (21) Appl. No.: 09/408,132 3435-3438. (22) Filed: Sep. 30, 1999 Grandjean et al., Tetrahedron Letters, (1994), 35(21), 3529-3530. Related U.S. Application Data Thompson et al., Tetrahedron Letters, (1995), 36(49), (60) Provisional application No. 60/102,643, filed on Oct. 1, 8937-8940. 1998. Ihara et al., Tetrahedron, (1995), 51(36), 9873–9890. Int. Cl." ...................... Bunnage and Nicolaou, Angew. Chem. Int. Ed. Engl., (51) - - - - - C07C 5700; CO7C 1/207; (1996), 35(10), 1110–1112. C07C 13/04; CO7C 69/708; CO7C 59/13; Carl Bernard Ziegler, Jr., Syhthesis and Mechanistic Studies C07C 67/31; CO7C 51/363 of Polyunsaturated Fatty Acid Hydroperoxides Involving a (52) U.S. -
United States Patent to 11 4,011,062 Demchak Et Al
United States Patent to 11 4,011,062 Demchak et al. (45 Mar. 8, 1977 (54) NOVEL COMPOSITIONS CONTAINING 3,389,185 6/1968 Bohm et al. ..................... 71/122 X ACETYLENC. GLYCOLSAFENERS FOR 3,857,692 12/1974 Feeny .............................. 71/122 X SPRING WHEAT 3,935,000 111976 Fischer .............................. 71/92 X (75) Inventors: Richard Joseph Demchak; David Lasilla Whitehead, both of Trenton, Primary Examiner-Joseph Paul Brust N.J. Attorney, Agent, or Firm-Harry H. Kline 73) Assignee: American Cyanamid Company, Stamford, Conn. 57 ABSTRACT 22 Filed: Sept. 9, 1975 Novel composition comprising in combination a 1,2- dialkwl-3,5-diphenvlpyrazolium salt and an acetylenic (21)21 Appl. No.:NO. 611,748 glycolyl-3,5-diphenylpy safener, and a method of utilizing said composiyient 52 U.S. Cl. ........................................ 71192; 71186 tion for the selective postemergence control of wild (51 int. Cl............................................ A01N 9/22 oats in spring wheat, whereby the said safener mini 58) Field of Search ................................ 71/92, 122 mizes the phytotoxic damage frequently caused by said 56) References Cited 1,2-dialkyl-3,5-diphenylpyrazolium salt. UNITED STATES PATENTS 2,997,447 8/1961 Russell et al. ................. 260/635 X 12 Claims, No Drawings 401 1,062 1 2 Surprisingly, it has been found that certain acetylenic NOVEL COMPOSITIONS CONTAINING glycols, when employed in admixture with the above ACETYLENIC GLYCOL SAFENERS FOR SPRING defined pyrazolium salts for the postemergence control WHEAT of wild oats in spring wheat, act as safeners or plant 5 protective agents and will minimize or eliminate the The present invention relates to a novel composition phytotoxic damage which can result from the use of comprising, in combination, a 1,2-dialkyl-3,5-diphenyl certain salts of formula (I) pyrazolium salts required to pyrazolium salt and an acetylenic glycol. -
1,2,4-Triazine-Accelerated Azide-Alkyne Cycloaddition and Synthesis of Metalloenzyme Inhibitors
University of Tennessee, Knoxville TRACE: Tennessee Research and Creative Exchange Doctoral Dissertations Graduate School 5-2013 1,2,4-Triazine-Accelerated Azide-Alkyne Cycloaddition and Synthesis of Metalloenzyme Inhibitors Belinda Shea Lady [email protected] Follow this and additional works at: https://trace.tennessee.edu/utk_graddiss Part of the Organic Chemistry Commons Recommended Citation Lady, Belinda Shea, "1,2,4-Triazine-Accelerated Azide-Alkyne Cycloaddition and Synthesis of Metalloenzyme Inhibitors. " PhD diss., University of Tennessee, 2013. https://trace.tennessee.edu/utk_graddiss/1750 This Dissertation is brought to you for free and open access by the Graduate School at TRACE: Tennessee Research and Creative Exchange. It has been accepted for inclusion in Doctoral Dissertations by an authorized administrator of TRACE: Tennessee Research and Creative Exchange. For more information, please contact [email protected]. To the Graduate Council: I am submitting herewith a dissertation written by Belinda Shea Lady entitled "1,2,4-Triazine- Accelerated Azide-Alkyne Cycloaddition and Synthesis of Metalloenzyme Inhibitors." I have examined the final electronic copy of this dissertation for form and content and recommend that it be accepted in partial fulfillment of the equirr ements for the degree of Doctor of Philosophy, with a major in Chemistry. Shane Foister, Major Professor We have read this dissertation and recommend its acceptance: Michael D. Best, Jimmy W. Mays, Thomas A. Zawodzinski Accepted for the Council: Carolyn R. Hodges Vice Provost and Dean of the Graduate School (Original signatures are on file with official studentecor r ds.) 1,2,4-Triazine-Accelerated Azide-Alkyne Cycloaddition and Synthesis of Metalloenzyme Inhibitors A Dissertation Presented for the Doctor of Philosophy Degree The University of Tennessee, Knoxville Belinda Shea Lady May 2013 Copyright © 2013 Belinda Shea Lady All rights reserved.