Density and Porosity of the Upper Lunar Crust from Combined Analysis of Gravity and Topography Data – Evaluation of the Interior Structure of Impact Basins
Total Page:16
File Type:pdf, Size:1020Kb
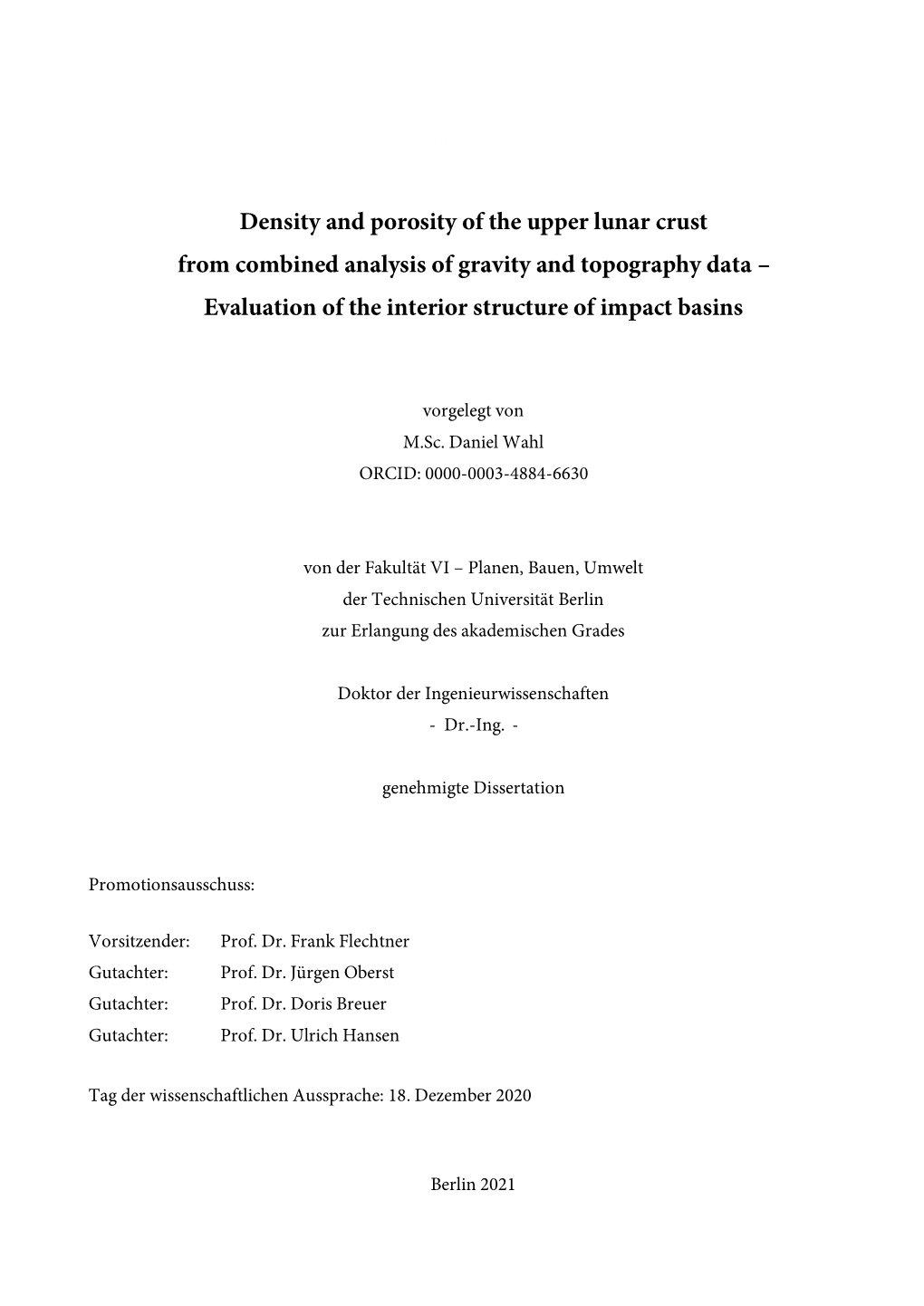
Load more
Recommended publications
-
Festskrift Til Harald Føsker Ellen Ekhaugen, Egil Larsen Og Fredrik Sjøli (Red.)
View metadata, citation and similar papers at core.ac.uk brought to you by CORE provided by NORA - Norwegian Open Research Archives Festskrift til Harald Føsker Ellen Ekhaugen, Egil Larsen og Fredrik Sjøli (red.) Kriminalomsorgens utdanningssenter, april 2014 1 Utgitt av Kriminalomsorgens utdanningssenter KRUS, 2014. Manusbearbeidelse og layout: Ottar Evensen og Maja Sørnes, KRUS Alle fotografier er gjengitt med artikkelforfatternes samtykke. ISBN trykket: 978-82-8257-033-6 ISBN pdf: 978-82-8257-034-3 Trykket hos 07-gruppen 2 Harald Føsker født 24. mai 1944 på Hamar Foto: Sturlason 3 BIDRAGSYTERE Balder, Jørgen – fhv uddannelseschef, KUC, Danmark Brucker, Hans-Jørgen – direktør, KRUS Five, Suzanne – seniorrådgiver, Kriminalomsorgsdirektoratet (KDI) Fridhov, Inger Marie – seniorrådgiver, Justisdepartementet Gislason, Gudmundur – fengselsdirektør/skoleleder, Island Hammerlin, Yngve – forsker, KRUS Hornslien, Anders - venn Johnsen, Berit – forskningsleder, KRUS Kornienko, Gennadij – generaldirektør, Den føderale russiske kriminalomsorgstjeneste Kristoffersen, Ragnar – forsker, KRUS Kvernvik Nilsen, Arne fengselsleder, Kriminalomsorgen Region Sør Larsen, Egil dekan, KRUS Lund-Isaksen, Erik – fhv. ekspedisjonssjef Nor, Susanne – HR direktør, Kriminalomsorgsdirektoratet (KDI) Orban, Franck – forsker, KRUS Porporino, Frank – Ph.D. Board Member ICPA Rentzmann, William – fhv. generaldirektør Kriminalforsorgen i Danmark Råen, Tore – friomsorgsleder i Nordtrøndelag Storberget, Knut – stortingsrepresentant og tidl. justisminister Vollan, Marianne -
Glossary Glossary
Glossary Glossary Albedo A measure of an object’s reflectivity. A pure white reflecting surface has an albedo of 1.0 (100%). A pitch-black, nonreflecting surface has an albedo of 0.0. The Moon is a fairly dark object with a combined albedo of 0.07 (reflecting 7% of the sunlight that falls upon it). The albedo range of the lunar maria is between 0.05 and 0.08. The brighter highlands have an albedo range from 0.09 to 0.15. Anorthosite Rocks rich in the mineral feldspar, making up much of the Moon’s bright highland regions. Aperture The diameter of a telescope’s objective lens or primary mirror. Apogee The point in the Moon’s orbit where it is furthest from the Earth. At apogee, the Moon can reach a maximum distance of 406,700 km from the Earth. Apollo The manned lunar program of the United States. Between July 1969 and December 1972, six Apollo missions landed on the Moon, allowing a total of 12 astronauts to explore its surface. Asteroid A minor planet. A large solid body of rock in orbit around the Sun. Banded crater A crater that displays dusky linear tracts on its inner walls and/or floor. 250 Basalt A dark, fine-grained volcanic rock, low in silicon, with a low viscosity. Basaltic material fills many of the Moon’s major basins, especially on the near side. Glossary Basin A very large circular impact structure (usually comprising multiple concentric rings) that usually displays some degree of flooding with lava. The largest and most conspicuous lava- flooded basins on the Moon are found on the near side, and most are filled to their outer edges with mare basalts. -
Evidence for Thermal-Stress-Induced Rockfalls on Mars Impact Crater Slopes
Icarus 342 (2020) 113503 Contents lists available at ScienceDirect Icarus journal homepage: www.elsevier.com/locate/icarus Evidence for thermal-stress-induced rockfalls on Mars impact crater slopes P.-A. Tesson a,b,*, S.J. Conway b, N. Mangold b, J. Ciazela a, S.R. Lewis c, D. M�ege a a Space Research Centre, Polish Academy of Science, Wrocław, Poland b Laboratoire de Plan�etologie et G�eodynamique UMR 6112, CNRS, Nantes, France c School of Physical Sciences, The Open University, Walton Hall, Milton Keynes MK7 6AA, UK ARTICLE INFO ABSTRACT Keywords: Here we study rocks falling from exposed outcrops of bedrock, which have left tracks on the slope over which Mars, surface they have bounced and/or rolled, in fresh impact craters (1–10 km in diameter) on Mars. The presence of these Thermal stress tracks shows that these rocks have fallen relatively recently because aeolian processes are known to infill Ices topographic lows over time. Mapping of rockfall tracks indicate trends in frequency with orientation, which in Solar radiation � � turn depend on the latitudinal position of the crater. Craters in the equatorial belt (between 15 N and 15 S) Weathering exhibit higher frequencies of rockfall on their north-south oriented slopes compared to their east-west ones. � Craters >15 N/S have notably higher frequencies on their equator-facing slopes as opposed to the other ori entations. We computed solar radiation on the surface of crater slopes to compare insolation patterns with the spatial distribution of rockfalls, and found statistically significant correlations between maximum diurnal inso lation and rockfall frequency. -
Martian Crater Morphology
ANALYSIS OF THE DEPTH-DIAMETER RELATIONSHIP OF MARTIAN CRATERS A Capstone Experience Thesis Presented by Jared Howenstine Completion Date: May 2006 Approved By: Professor M. Darby Dyar, Astronomy Professor Christopher Condit, Geology Professor Judith Young, Astronomy Abstract Title: Analysis of the Depth-Diameter Relationship of Martian Craters Author: Jared Howenstine, Astronomy Approved By: Judith Young, Astronomy Approved By: M. Darby Dyar, Astronomy Approved By: Christopher Condit, Geology CE Type: Departmental Honors Project Using a gridded version of maritan topography with the computer program Gridview, this project studied the depth-diameter relationship of martian impact craters. The work encompasses 361 profiles of impacts with diameters larger than 15 kilometers and is a continuation of work that was started at the Lunar and Planetary Institute in Houston, Texas under the guidance of Dr. Walter S. Keifer. Using the most ‘pristine,’ or deepest craters in the data a depth-diameter relationship was determined: d = 0.610D 0.327 , where d is the depth of the crater and D is the diameter of the crater, both in kilometers. This relationship can then be used to estimate the theoretical depth of any impact radius, and therefore can be used to estimate the pristine shape of the crater. With a depth-diameter ratio for a particular crater, the measured depth can then be compared to this theoretical value and an estimate of the amount of material within the crater, or fill, can then be calculated. The data includes 140 named impact craters, 3 basins, and 218 other impacts. The named data encompasses all named impact structures of greater than 100 kilometers in diameter. -
A La Torre Aaker Aalbers Aaldert Aarmour Aaron
A LA TORRE ABDIE ABLEMAN ABRAMOWITCH AAKER ABE ABLES ABRAMOWITZ AALBERS ABEE ABLETSON ABRAMOWSKY AALDERT ABEEL ABLETT ABRAMS AARMOUR ABEELS ABLEY ABRAMSEN AARON ABEKE ABLI ABRAMSKI AARONS ABEKEN ABLITT ABRAMSON AARONSON ABEKING ABLOTT ABRAMZON AASEN ABEL ABNER ABRASHKIN ABAD ABELA ABNETT ABRELL ABADAM ABELE ABNEY ABREU ABADIE ABELER ABORDEAN ABREY ABALOS ABELES ABORDENE ABRIANI ABARCA ABELI ABOT ABRIL ABATE ABELIN ABOTS ABRLI ABB ABELL ABOTSON ABRUZZO ABBA ABELLA ABOTT ABSALOM ABBARCROMBIE ABELLE ABOTTS ABSALON ABBAS ABELLS ABOTTSON ABSHALON ABBAT ABELMAN ABRAHAM ABSHER ABBATE ABELS ABRAHAMER ABSHIRE ABBATIELLO ABELSON ABRAHAMI ABSOLEM ABBATT ABEMA ABRAHAMIAN ABSOLOM ABBAY ABEN ABRAHAMOF ABSOLON ABBAYE ABENDROTH ABRAHAMOFF ABSON ABBAYS ABER ABRAHAMOV ABSTON ABBDIE ABERCROMBIE ABRAHAMOVITZ ABT ABBE ABERCROMBY ABRAHAMOWICZ ABTS ABBEKE ABERCRUMBIE ABRAHAMS ABURN ABBEL ABERCRUMBY ABRAHAMS ABY ABBELD ABERCRUMMY ABRAHAMSEN ABYRCRUMBIE ABBELL ABERDEAN ABRAHAMSOHN ABYRCRUMBY ABBELLS ABERDEEN ABRAHAMSON AC ABBELS ABERDEIN ABRAHAMSSON ACASTER ABBEMA ABERDENE ABRAHAMY ACCA ABBEN ABERG ABRAHM ACCARDI ABBERCROMBIE ABERLE ABRAHMOV ACCARDO ABBERCROMMIE ABERLI ABRAHMOVICI ACE ABBERCRUMBIE ABERLIN ABRAHMS ACERO ABBERDENE ABERNATHY ABRAHMSON ACESTER ABBERDINE ABERNETHY ABRAM ACETO ABBERLEY ABERT ABRAMCHIK ACEVEDO ABBETT ABEYTA ABRAMCIK ACEVES ABBEY ABHERCROMBIE ABRAMI ACHARD ABBIE ABHIRCROMBIE ABRAMIN ACHENBACH ABBING ABIRCOMBIE ABRAMINO ACHENSON ABBIRCROMBIE ABIRCROMBIE ABRAMO ACHERSON ABBIRCROMBY ABIRCROMBY ABRAMOF ACHESON ABBIRCRUMMY ABIRCROMMBIE ABRAMOFF -
Sky and Telescope
SkyandTelescope.com The Lunar 100 By Charles A. Wood Just about every telescope user is familiar with French comet hunter Charles Messier's catalog of fuzzy objects. Messier's 18th-century listing of 109 galaxies, clusters, and nebulae contains some of the largest, brightest, and most visually interesting deep-sky treasures visible from the Northern Hemisphere. Little wonder that observing all the M objects is regarded as a virtual rite of passage for amateur astronomers. But the night sky offers an object that is larger, brighter, and more visually captivating than anything on Messier's list: the Moon. Yet many backyard astronomers never go beyond the astro-tourist stage to acquire the knowledge and understanding necessary to really appreciate what they're looking at, and how magnificent and amazing it truly is. Perhaps this is because after they identify a few of the Moon's most conspicuous features, many amateurs don't know where Many Lunar 100 selections are plainly visible in this image of the full Moon, while others require to look next. a more detailed view, different illumination, or favorable libration. North is up. S&T: Gary The Lunar 100 list is an attempt to provide Moon lovers with Seronik something akin to what deep-sky observers enjoy with the Messier catalog: a selection of telescopic sights to ignite interest and enhance understanding. Presented here is a selection of the Moon's 100 most interesting regions, craters, basins, mountains, rilles, and domes. I challenge observers to find and observe them all and, more important, to consider what each feature tells us about lunar and Earth history. -
Effect of Target Properties on the Impact Cratering Process
WORKSHOP PROGRAM AND ABSTRACTS LPI Contribution No. 1360 BRIDGING THE GAP II: EFFECT OF TARGET PROPERTIES ON THE IMPACT CRATERING PROCESS September 22–26, 2007 Saint-Hubert, Canada SPONSORS Canadian Space Agency Lunar and Planetary Institute Barringer Crater Company NASA Planetary Geology and Geophysics Program CONVENERS Robert Herrick, University of Alaska Fairbanks Gordon Osinski, Canadian Space Agency Elisabetta Pierazzo, Planetary Science Institute SCIENTIFIC ORGANIZING COMMITTEE Mark Burchell, University of Kent Gareth Collins, Imperial College London Michael Dence, Canadian Academy of Science Kevin Housen, Boeing Corporation Jay Melosh, University of Arizona John Spray, University of New Brunswick Lunar and Planetary Institute 3600 Bay Area Boulevard Houston TX 77058-1113 LPI Contribution No. 1360 Compiled in 2007 by LUNAR AND PLANETARY INSTITUTE The Institute is operated by the Universities Space Research Association under Agreement No. NCC5-679 issued through the Solar System Exploration Division of the National Aeronautics and Space Administration. Any opinions, findings, and conclusions or recommendations expressed in this volume are those of the author(s) and do not necessarily reflect the views of the National Aeronautics and Space Administration. Material in this volume may be copied without restraint for library, abstract service, education, or personal research purposes; however, republication of any paper or portion thereof requires the written permission of the authors as well as the appropriate acknowledgment of this publication. Abstracts in this volume may be cited as Author A. B. (2007) Title of abstract. In Bridging the Gap II: Effect of Target Properties on the Impact Cratering Process, p. XX. LPI Contribution No. 1360, Lunar and Planetary Institute, Houston. -
Analysis of Polarimetric Mini-SAR and Mini-RF Datasets for Surface Characterization and Crater Delineation on Moon †
Proceeding Paper Analysis of Polarimetric Mini-SAR and Mini-RF Datasets for Surface Characterization and Crater Delineation on Moon † Himanshu Kumari and Ashutosh Bhardwaj * Photogrammetry and Remote Sensing Department, Indian Institute of Remote Sensing, Dehradun 248001, India; [email protected] * Correspondence: [email protected]; Tel.: +91-9410319433 † Presented at the 3rd International Electronic Conference on Atmospheric Sciences, 16–30 November 2020; Available online: https://ecas2020.sciforum.net/. Abstract: The hybrid polarimetric architecture of Mini-SAR and Mini-RF onboard Indian Chan- drayaan-1 and LRO missions were the first to acquire shadowed polar images of the Lunar surface. This study aimed to characterize the surface properties of Lunar polar and non-polar regions con- taining Haworth, Nobile, Gioja, an unnamed crater, Arago, and Moltke craters and delineate the crater boundaries using a newly emerged approach. The Terrain Mapping Camera (TMC) data of Chandrayaan-1 was found useful for the detection and extraction of precise boundaries of the cra- ters using the ArcGIS Crater tool. The Stokes child parameters estimated from radar backscatter like the degree of polarization (m), the relative phase (δ), Poincare ellipticity (χ) along with the Circular Polarization Ratio (CPR), and decomposition techniques, were used to study the surface attributes of craters. The Eigenvectors and Eigenvalues used to measure entropy and mean alpha showed distinct types of scattering, thus its comparison with m-δ, m-χ gave a profound conclusion to the lunar surface. The dominance of surface scattering confirmed the roughness of rugged material. The results showed the CPR associated with the presence of water ice as well as a dihedral reflection inside the polar craters. -
The Pancam Instrument for the Exomars Rover
ASTROBIOLOGY ExoMars Rover Mission Volume 17, Numbers 6 and 7, 2017 Mary Ann Liebert, Inc. DOI: 10.1089/ast.2016.1548 The PanCam Instrument for the ExoMars Rover A.J. Coates,1,2 R. Jaumann,3 A.D. Griffiths,1,2 C.E. Leff,1,2 N. Schmitz,3 J.-L. Josset,4 G. Paar,5 M. Gunn,6 E. Hauber,3 C.R. Cousins,7 R.E. Cross,6 P. Grindrod,2,8 J.C. Bridges,9 M. Balme,10 S. Gupta,11 I.A. Crawford,2,8 P. Irwin,12 R. Stabbins,1,2 D. Tirsch,3 J.L. Vago,13 T. Theodorou,1,2 M. Caballo-Perucha,5 G.R. Osinski,14 and the PanCam Team Abstract The scientific objectives of the ExoMars rover are designed to answer several key questions in the search for life on Mars. In particular, the unique subsurface drill will address some of these, such as the possible existence and stability of subsurface organics. PanCam will establish the surface geological and morphological context for the mission, working in collaboration with other context instruments. Here, we describe the PanCam scientific objectives in geology, atmospheric science, and 3-D vision. We discuss the design of PanCam, which includes a stereo pair of Wide Angle Cameras (WACs), each of which has an 11-position filter wheel and a High Resolution Camera (HRC) for high-resolution investigations of rock texture at a distance. The cameras and electronics are housed in an optical bench that provides the mechanical interface to the rover mast and a planetary protection barrier. -
March 21–25, 2016
FORTY-SEVENTH LUNAR AND PLANETARY SCIENCE CONFERENCE PROGRAM OF TECHNICAL SESSIONS MARCH 21–25, 2016 The Woodlands Waterway Marriott Hotel and Convention Center The Woodlands, Texas INSTITUTIONAL SUPPORT Universities Space Research Association Lunar and Planetary Institute National Aeronautics and Space Administration CONFERENCE CO-CHAIRS Stephen Mackwell, Lunar and Planetary Institute Eileen Stansbery, NASA Johnson Space Center PROGRAM COMMITTEE CHAIRS David Draper, NASA Johnson Space Center Walter Kiefer, Lunar and Planetary Institute PROGRAM COMMITTEE P. Doug Archer, NASA Johnson Space Center Nicolas LeCorvec, Lunar and Planetary Institute Katherine Bermingham, University of Maryland Yo Matsubara, Smithsonian Institute Janice Bishop, SETI and NASA Ames Research Center Francis McCubbin, NASA Johnson Space Center Jeremy Boyce, University of California, Los Angeles Andrew Needham, Carnegie Institution of Washington Lisa Danielson, NASA Johnson Space Center Lan-Anh Nguyen, NASA Johnson Space Center Deepak Dhingra, University of Idaho Paul Niles, NASA Johnson Space Center Stephen Elardo, Carnegie Institution of Washington Dorothy Oehler, NASA Johnson Space Center Marc Fries, NASA Johnson Space Center D. Alex Patthoff, Jet Propulsion Laboratory Cyrena Goodrich, Lunar and Planetary Institute Elizabeth Rampe, Aerodyne Industries, Jacobs JETS at John Gruener, NASA Johnson Space Center NASA Johnson Space Center Justin Hagerty, U.S. Geological Survey Carol Raymond, Jet Propulsion Laboratory Lindsay Hays, Jet Propulsion Laboratory Paul Schenk, -
Impact Melt Flows on the Moon: Observations and Models
EPSC Abstracts Vol. 13, EPSC-DPS2019-1067-1, 2019 EPSC-DPS Joint Meeting 2019 c Author(s) 2019. CC Attribution 4.0 license. Impact melt flows on the Moon: observations and models Natalia Artemieva (1,2), Veronica Bray (3) (1) Planetary Science Institute, Arizona, USA, (2) Institute for Dynamics of Geospheres ([email protected]) , (3) Lunar and Planetary Laboratory, University of Arizona, USA Abstract topography. Our numerical modeling explores this supposition. We compare modeling results with observed melt flows in the extended ejecta blanket of the Pierazzo crater on the Moon. Surface flow dynamics of ballistically deposited melts depends on its viscosity, deposition velocity (i.e., distance from the parent crater), local topography, and the amount of entrained cold materials. 1. Introduction This work concentrates on the analysis of Pierazzo crater (9.2 km in diameter) located on the far side of the Moon (259.7E, 3.25N) [1]. This crater has extensive LRO Narrow Angle Camera (NAC) coverage and a visible ray system extending beyond 450 km from the crater rim - suggesting a relatively young age. We analyse and model melt flows within the discontinuous ejecta at distances 11-40 km from the crater rim. 1.1 Mapping LROC images extending ~ 40km from the Pierazzo crater rim were mosaicked and melt flows and ponds mapped (Fig 1A). The largest flows show clear melt- like morphology: cooling cracks, channelling, lobate toe and pooling in low topography (Fig 1B/C). Fig. 1: A) Simplified mapping of [1] to show only the Fractal dimensions of several flows were recorded (D lobate flows present around Pierazzo crater (black). -
Curt Teich Postcard Archives Towns and Cities
Curt Teich Postcard Archives Towns and Cities Alaska Aialik Bay Alaska Highway Alcan Highway Anchorage Arctic Auk Lake Cape Prince of Wales Castle Rock Chilkoot Pass Columbia Glacier Cook Inlet Copper River Cordova Curry Dawson Denali Denali National Park Eagle Fairbanks Five Finger Rapids Gastineau Channel Glacier Bay Glenn Highway Haines Harding Gateway Homer Hoonah Hurricane Gulch Inland Passage Inside Passage Isabel Pass Juneau Katmai National Monument Kenai Kenai Lake Kenai Peninsula Kenai River Kechikan Ketchikan Creek Kodiak Kodiak Island Kotzebue Lake Atlin Lake Bennett Latouche Lynn Canal Matanuska Valley McKinley Park Mendenhall Glacier Miles Canyon Montgomery Mount Blackburn Mount Dewey Mount McKinley Mount McKinley Park Mount O’Neal Mount Sanford Muir Glacier Nome North Slope Noyes Island Nushagak Opelika Palmer Petersburg Pribilof Island Resurrection Bay Richardson Highway Rocy Point St. Michael Sawtooth Mountain Sentinal Island Seward Sitka Sitka National Park Skagway Southeastern Alaska Stikine Rier Sulzer Summit Swift Current Taku Glacier Taku Inlet Taku Lodge Tanana Tanana River Tok Tunnel Mountain Valdez White Pass Whitehorse Wrangell Wrangell Narrow Yukon Yukon River General Views—no specific location Alabama Albany Albertville Alexander City Andalusia Anniston Ashford Athens Attalla Auburn Batesville Bessemer Birmingham Blue Lake Blue Springs Boaz Bobler’s Creek Boyles Brewton Bridgeport Camden Camp Hill Camp Rucker Carbon Hill Castleberry Centerville Centre Chapman Chattahoochee Valley Cheaha State Park Choctaw County